Chromosome condensation and compaction is nothing short than awe-inspiring, amazing evidence of setup by a supreme intelligence.
https://reasonandscience.catsboard.com/t2086-chromosome-condensation-amazing-evidence-of-design
Imagine trying to stuff about 10,000 miles of spaghetti inside a basketball. Then, if that was not difficult enough, attempt to find a unique one-inch segment of pasta from the middle of this mess, or try to duplicate, untangle and separate individual strings to opposite ends. This simple analogy illustrates some of the daunting tasks associated with the transcription, repair and replication of the nearly 2 meters of DNA that is packaged into the confines of a tiny eukaryotic nucleus. The solution to each of these problems lies in the assembly of the eukaryotic genome into chromatin, a structural polymer that not only solves the basic packaging problem but also provides a dynamic platform that controls all DNA-mediated processes within the nucleus.
Wrapping of DNA around a nucleosome core compacts the DNA length about sevenfold. The overall compaction in a chromosome, however, is greater than 10,000-fold—ample evidence for even higher orders of structural organization. Nucleosome cores are further organized into a structure called the 30 nm fiber. This packing requires one molecule of histone H1 per nucleosome core. The 30 nm fiber—a second level of chromatin organization—provides an approximately 100-fold compaction of the DNA. The next level of higher organization depends on nuclear scaffold proteins. The scaffold-associated regions are separated by loops of DNA. The DNA in a loop may contain a set of related genes. In Drosophila, for example, complete sets of histone-coding genes seem to cluster together in loops that are bounded by scaffold attachment sites. Additional layers of organization in eukaryotic chromosomes, each dramatically enhancing the degree of compaction.
Packing ratio - the length of DNA divided by the length into which it is packaged
The shortest human chromosome contains 4.6 x 107 bp of DNA (about 10 times the genome size of E. coli). This is equivalent to 14,000 µm of extended DNA, or about 2 meters. In its most condensed state during mitosis, the chromosome is about 2 µm long. This gives a packing ratio of 7000 (14,000/2).
To achieve the overall packing ratio, DNA is not packaged directly into the final structure of chromatin. Instead, it contains several hierarchies of organization.
The first level of packing is achieved by the winding of DNA around a protein core to produce a "bead-like" structure called a nucleosome. This gives a packing ratio of about 6. This structure is invariant in both the euchromatin and heterochromatin of all chromosomes.
The second level of packing is the coiling of beads in a helical structure called the 30 nm fiber that is found in both interphase chromatin and mitotic chromosomes. This structure increases the packing ratio to about 40.
The final packaging occurs when the fiber is organized in loops, scaffolds, and domains that give a final packing ratio of about 1000 in interphase chromosomes and about 7,000 in mitotic chromosomes.
That's an amazing change, from a ratio of 6 to 7.000 !!
Evolution, or design ??
Imagine trying to stuff about 10,000 miles of spaghetti inside a basketball. Then, if that was not difficult enough, attempt to find a unique one inch segment of pasta from the middle of this mess, or try to duplicate, untangle and separate individual strings to opposite ends. This simple analogy illustrates some of the daunting tasks associated with the transcription, repair and replication of the nearly 2 meters of DNA that is packaged into the confines of a tiny eukaryotic nucleus. The solution to each of these problems lies in the assembly of the eukaryotic genome into chromatin, a structural polymer that not only solves the basic packaging problem, but also provides a dynamic platform that controls all DNA-mediated processes within the nucleus. 6
Wrapping of DNA around a nucleosome core compacts the DNA length about sevenfold. The overall compaction in a chromosome, however, is greater than 10,000-fold—ample evidence for even higher orders of structural organization. In chromosomes isolated by very gentle methods, nucleosome cores seem to be organized into a structure called the 30 nm fiber.

The 30 nm fiber, a higher-order organization of nucleosomes.
(a) Schematic illustration of the probable structure of the fiber, showing nucleosome packing.
(b) Electron micrograph.
This packing requires one molecule of histone H1 per nucleosome core. Organization into 30 nm fibers does not extend over the entire chromosome but is punctuated by regions bound by sequence-specific (nonhistone) DNA-binding proteins. The 30 nm structure also seems to depend on the transcriptional activity of the particular region of DNA. Regions in which genes are being transcribed are apparently in a less-ordered state that contains little if any, histone H1.
The histone modifications do not disappear at cell division or during meiosis, and thus they become part of the information transmitted from one generation to the next in all eukaryotic organisms.
The 30 nm fiber—a second level of chromatin organization—provides an approximately 100-fold compaction of the DNA. Certain regions of DNA seem to associate with a nuclear scaffold. The scaffold-associated regions are separated by loops of DNA. The DNA in a loop may contain a set of related genes. In Drosophila, for example, complete sets of histone-coding genes seem to cluster together in loops that are bounded by scaffold attachment sites.

Loops of chromosomal DNA attached to a nuclear scaffold.
The DNA in the loops is packaged as 30 nm fibers, so the loops are the next level of organization. Loops often contain groups of genes with related functions. Complete sets of histone-coding genes, as shown in this schematic illustration, seem to be clustered in loops of this kind. Unlike most genes, histone genes occur in multiple copies in many eukaryotic genomes.
The scaffold itself may contain several proteins, notably large amounts of histone H1 (located in the interior of the fiber) and topoisomerase II. The presence of topoisomerase II further emphasizes the relationship between DNA underwinding and chromatin structure. Topoisomerase II is so important to the maintenance of chromatin structure that inhibitors of this enzyme can kill rapidly dividing cells. Evidence exists for additional layers of organization in eukaryotic chromosomes, each dramatically enhancing the degree of compaction.

Compaction of DNA in a eukaryotic chromosome.
This model shows the levels of organization that could provide the observed degree of DNA compaction in the chromosomes of eukaryotes. The levels take the form of coils upon coils. In cells, the higher-order structures (above
the 30 nm fibers) are unlikely to be as uniform as depicted here.
DNA compaction in eukaryotic chromosomes is likely to involve coils upon coils upon coils . . .
Every second, the cells constituting our bodies are replaced through cell division.An adult human consists of about 50,000 billion cells, 1% of which die and are replaced by cell division every day. In order to ensure cell survival and controlled growth of these new cells, the genetic information, stored in DNA molecules, must first be correctly copied and then accurately distributed during cell division. Moreover, to fully ascertain that the new cells will contain the same genetic information as the parental cells, any damage to the DNA, which is organised into several chromosomes, must be repaired. 3
Quite a bit is known about two of these complexes. One of them, cohesin, keeps the DNA copies together such that they do not separate too early; while the other, condensin, makes the chromosomes more compact, making the separation easier.
Prophase
During the first stage of mitosis, that of prophase, the duplicated chromosomes are prepared for segregation and the mitotic machinery is assembled.
Formation of the Mitotic Chromosome
The nucleus of an interphase cell contains tremendous lengths of chromatin fibers. The extended state of interphase chromatin is ideally suited for the processes of transcription and replication but not for segregation into two daughter cells. Before segregating its chromosomes, a cell converts them into much shorter, thicker structures by a remarkable process of chromosome compaction (or chromosome condensation), which occurs during early prophase

Research on chromosome compaction has focused on an abundant multiprotein complex called condensin.
Packing ratio - the length of DNA divided by the length into which it is packaged
The shortest human chromosome contains 4.6 x 107 bp of DNA (about 10 times the genome size of E. coli). This is equivalent to 14,000 µm of extended DNA, or about 2 meters. In its most condensed state during mitosis, the chromosome is about 2 µm long. This gives a packing ratio of 7000 (14,000/2).
To achieve the overall packing ratio, DNA is not packaged directly into the final structure of chromatin. Instead, it contains several hierarchies of organization.
The first level of packing is achieved by the winding of DNA around a protein core to produce a "bead-like" structure called a nucleosome. This gives a packing ratio of about 6. This structure is invariant in both the euchromatin and heterochromatin of all chromosomes.
The second level of packing is the coiling of beads in a helical structure called the 30 nm fiber that is found in both interphase chromatin and mitotic chromosomes. This structure increases the packing ratio to about 40.
The final packaging occurs when the fiber is organized in loops, scaffolds, and domains that give a final packing ratio of about 1000 in interphase chromosomes and about 7,000 in mitotic chromosomes.
That's an amazing change, from a ratio of 6 to 7.000 !!
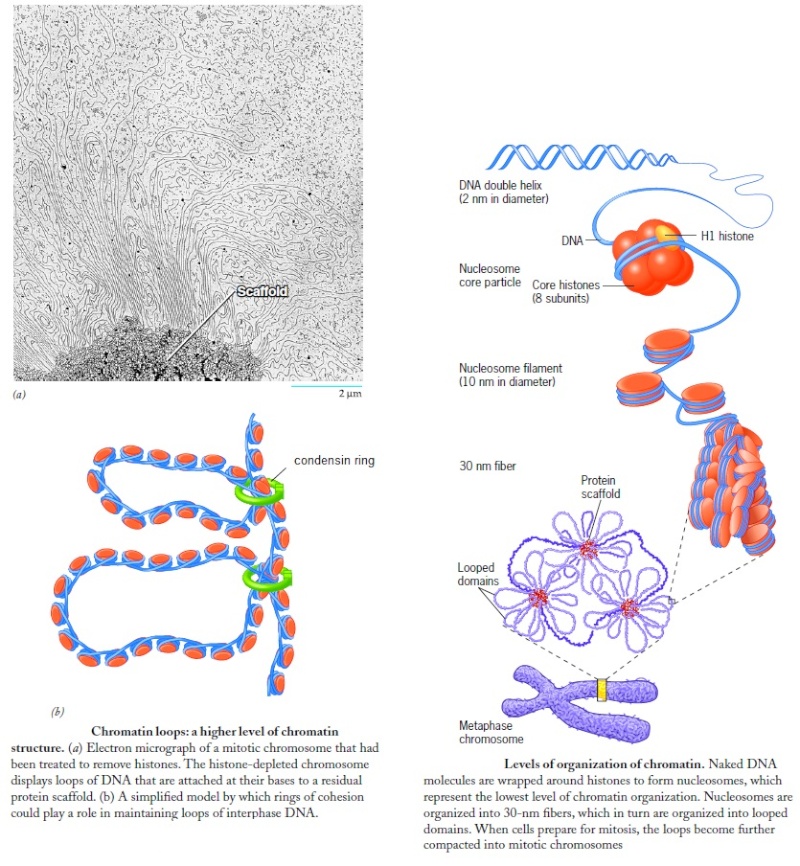
Squeezing DNA Into A Small Space
To fit 2 meters of DNA into a tiny nucleus is a monumental engineering feat. DNA is highly compacted yet has to be instantly available to rapidly make proteins in neurons with a momentary change of thought. This regulation is different in each type of cell. . It has been known for some time that the shape of proteins determines their function and the folding is very complex involving four levels of folding . Now it appears that the shape of the chromatin, also, determines function, with new secondary and tertiary structures discovered. 23
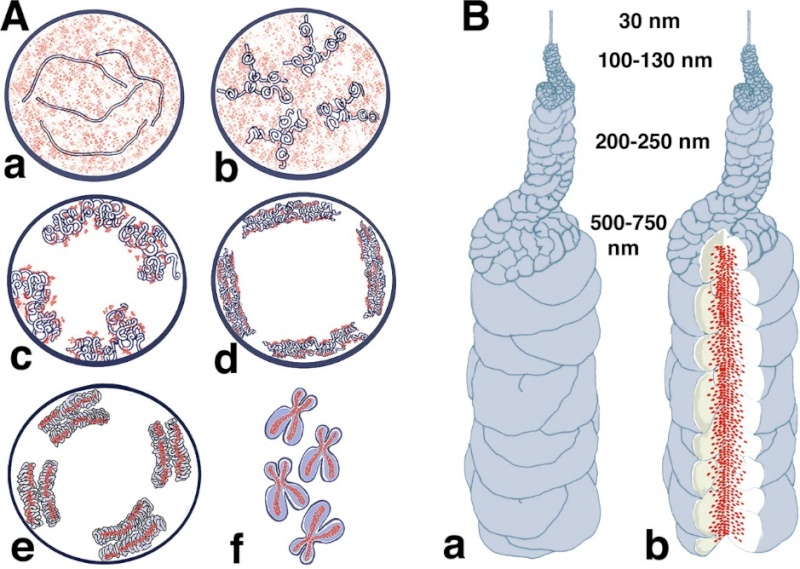
Models for chromosome condensation.
(A) Stages of condensation. Changes in large-scale chromatin folding (blue) versus SMC2 distribution (red) from early S (a), G2 (b), early prophase (c), middle prophase (d), late prophase (e), and metaphase (f). See text for details.
(B) “Hierarchical folding, axial glue” model of metaphase chromosome structure. (a) 30-nm fiber folds into 100–130-nm chromonema fiber, which folds into 200–250-nm middle prophase chromatid, which folds into 500–750-nm metaphase chromatid. Only one chromatid is shown here. (b) Axial condensin distribution (green) occupies approximately one-third of the chromatid diameter, acting as cross-linking “glue” to stabilize metaphase chromosome.
Condensins: universal organizers of chromosomes with diverse functions
Condensins are multisubunit protein complexes that play a fundamental role in the structural and functional organization of chromosomes in the three domains of life. It is a molecular machine that helps to condense and package chromosomes for cell replication. It is a five-subunit complex and is “the key molecular machine of chromosome condensation. 24
Condensin produces “super coils” of DNA, one of many steps in packing the delicate DNA strands into a hierarchy of coils that results in a densely-packed chromosome. “It is not entirely clear how the DNA is held in this supercoiled state,” they say, “but several studies suggest that the V-shaped arms of the condensin complex may loop and clamp the DNA in place.” This clamping is “rapid and reversible.” Scientists watching the process in both bacteria and humans are “showing that both vertebrate and bacterial condensins drive DNA compaction in an ATP-dependent fashion with a surprising level of co-operativity that was not fully appreciated.” The condensin molecules work as a team; if not enough condensin is around, nothing happens. These authors point out also that condensin is just one of many enzymes involved in chromosome formation. Think about how remarkable it is that during each cell division, the chromosomes are structured so reliably that they can be labeled and numbered under the microscope. “Our own proteomic analysis,” they claim, “has identified over 350 chromosome-associated proteins, so there is clearly more work to be done.” 6
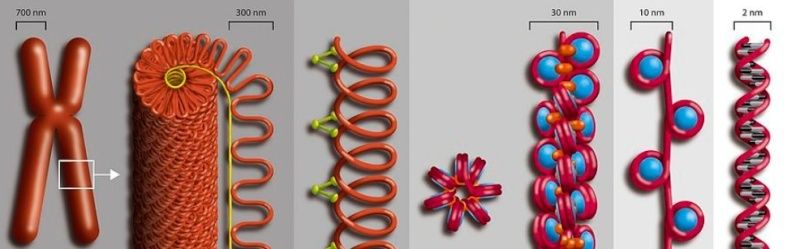
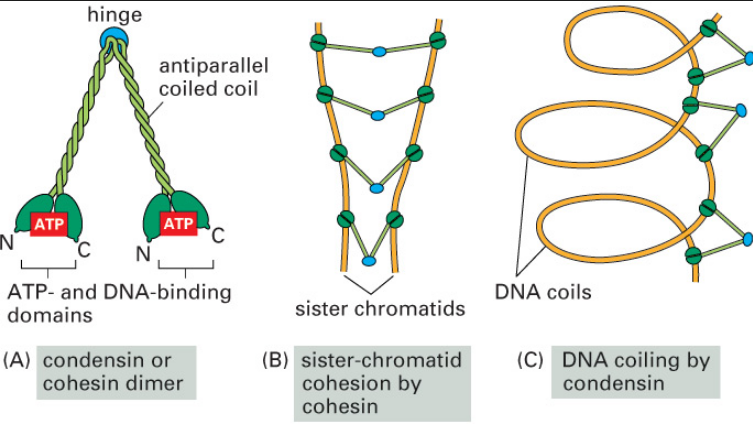
SMC proteins
SMC proteins represent a large family of ATPases that participate in many aspects of higher-order chromosome organization and dynamics. SMC stands for Structural Maintenance of Chromosomes.8
Eukaryotic SMCs
Eukaryotes have at least six SMC proteins in individual organisms, and they form three distinct heterodimers with specialized functions: A pair of SMC1 and SMC3 constitutes the core subunits of the cohesin complexes involved in sister chromatid cohesion. Likewise, a pair of SMC2 and SMC4 acts as the core of the condensin complexes implicated in chromosome condensation. A dimer composed of SMC5 and SMC6 functions as part of a yet-to-be-named complex implicated in DNA repair and checkpoint responses.
SMC proteins are conserved from bacteria to humans.
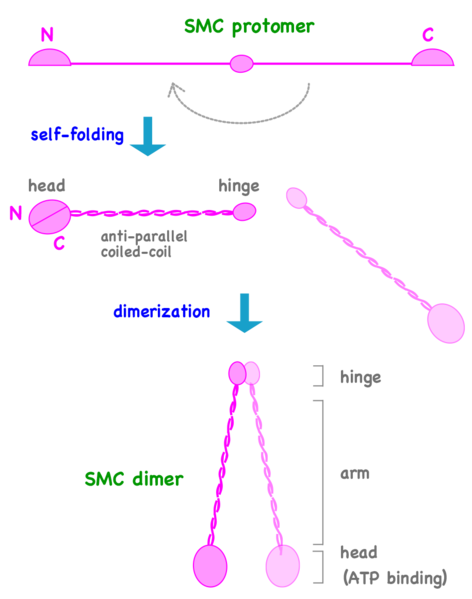
Can We Live Without SMCs? No!
The Structural Maintenance of Chromosomes (SMC) proteins are a family of chromosomal ATPases highly conserved among the three phyla of life. ... What do SMCs look like? The SMC proteins are large polypeptides, each spanning 1000-1500 amino acids. They form dimers in which two anti-parallel coiled-coil arms are connected by a flexible hinge. ... The distal end of each arm constitutes an ATP-binding domain.7
This family of proteins look like tweezers that can grab DNA and keep it under control during critical processes in the cell. They are important in holding sister chromatids together and separating them during cell division. Some are also “implicated in DNA repair and checkpoint responses.” They are also essential for the proper separation of chromosomes during gamete formation during meiotic cell division. So how do these miniature grappling hooks do all this? How do SMCs work? That is the million-dollar question in the field. Of particular interest is to understand how the two-armed structure - which is approximately 100 nm long when it’s open! - captures DNA, and how these interactions are modulated by ATP binding and hydrolysis. Condensin is able to introduce positive supercoils into DNA by using the energy of ATP hydrolysis.
Can we live without SMCs? No! Loss of any single SMC protein in budding yeast is lethal.
The short article by Gillespie and Hirano (Cold Spring Harbor Laboratories, NY) contains a diagram of what two sample SMC proteins look like. The SMC “superfamily” work in complexes with other molecules to accomplish these vital tasks. 9
Condensins are multisubunit protein complexes that play a fundamental role in the structural and functional organization of chromosomes in the three domains of life. 1
The chromosomal condensin complex is a major molecular effector of chromosome condensation and segregation in diverse organisms ranging from bacteria to humans. Condensin is a large, evolutionarily conserved, multisubunit protein assembly composed of dimers of the structural maintenance of chromosomes (SMC) family of ATPases, clasped into topologically closed rings by accessory subunits. Condensin binds to DNA dynamically, in a poorly understood cycle of ATP-modulated conformational changes, and exhibits the ability to positively supercoil DNA. During mitosis, condensin is phosphorylated by the cyclin-dependent kinase (CDK), Polo and Aurora B kinases in a manner that correlates with changes in its localisation, dynamics and supercoiling activity.
Condensin helps configure duplicated chromosomes for separation
At the end of S phase, the immensely long DNA molecules of the sister chromatids are tangled in a mass of partially catenated DNA and proteins. Any attempt to pull the sisters apart in this state would undoubtedly lead to breaks in the chromosomes. To avoid this disaster, the cell devotes a great deal of energy in early mitosis to gradually reorganizing the sister chromatids into relatively short, distinct structures that can be pulled apart more easily in anaphase. These chromosomal changes involve two processes: chromosome condensation, in which the chromatids are dramatically compacted; and sister-chromatid resolution, whereby the two sisters are resolved into distinct, separable units

Resolution results from the decatenation of the sister DNAs, accompanied by the partial removal of cohesin molecules along the chromosome arms.
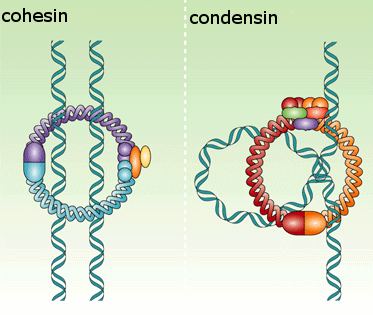
As a result, when the cell reaches metaphase, the sister chromatids appear in the microscope as compact, rod-like structures that are joined tightly at their centromeric regions and only loosely along their arms.The condensation and resolution of sister chromatids depends, at least in part, on a five-subunit protein complex called condensin. Condensin structure is related to that of the cohesin complex that holds sister chromatids together
It contains two SMC subunits like those of cohesin, plus three non-SMC subunits
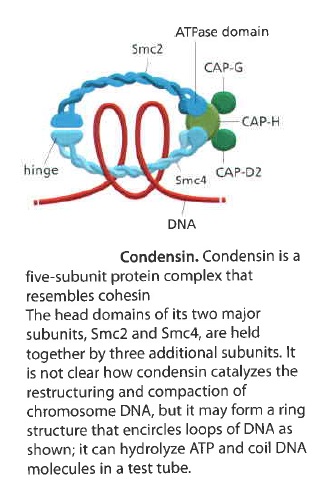
Condensin may form a ring-like structure that somehow uses the energy provided byATP hydrolysis to promote the compaction and resolution of sister chromatids. Condensin is able to change the coiling of DNA molecules in a test tube, and this coiling activity is thought to be important for chromosome condensation during mitosis. Interestingly, phosphorylation of condensin subunits by M-Cdk stimulates this coiling activity, providing one mechanism by which M-Cdk may promote chromosome restructuring in early mitosis.
There is third, less well understood, protein complex, known as the Smc5/6 complex. 3 This protein complex was found to bind to locations on the DNA strand that the researchers had artificially damaged, suggesting that it is directly involved in the repair process. Moreover, the Smc5/6 complex also seems to be required for the disentanglement of undamaged chromosomes before cell division. If these tangles, which are a natural consequence of the DNA copying process, are left unresolved the chromosomes cannot be separated and sent to the two nascent daughter cells. Like in the repair process, the Smc5/6 complex appears to resolve these intertwines by direct interaction with the DNA molecules, but this process is differently regulated as compared to the function in repair.
"Evidence points to that the Smc5/6 complex work in two different pathways, one needed for repair and the other for untangling.
The Smc5/6 complex: more than repair?
Through its functions in chromosome replication, segregation, and repair, the Smc5/6 complex has a central role in the maintenance of genome stability. The complex is part of the family of structural maintenance of chromosome protein complexes that also includes cohesin and condensin. Mutations in any of these complexes disrupt chromosome segregation and render cells hypersensitive to different types of DNA damage. The chromosome mis-segregation phenotypes in cohesin and condensin mutants can be attributed to their functions in sister chromatid cohesion and chromosome condensation, respectively. Cohesin-dependent chromatid cohesion is also needed for DNA double-strand break repair, whereas condensin is required for repair of single-strand breaks. How Smc5/6 promotes chromosome stability is largely unknown. Accumulating data suggest that it prevents accumulation of aberrant DNA links between sister chromatids created during repair by homologous recombination. A long-standing idea is that it also has a role in the maintenance of nondamaged chromosomes. Here, we present an overview of the current knowledge of Smc5/6 and discuss a possible non-repair role of the complex. 4
The Chromosomal Association of the Smc5/6 Complex Depends on Cohesion and Predicts the Level of Sister Chromatid Entanglement 5
The cohesin complex, which is essential for sister chromatid cohesion and chromosome segregation, also inhibits resolution of sister chromatid intertwinings (SCIs) by the topoisomerase Top2. The cohesin-related Smc5/6 complex (Smc5/6) instead accumulates on chromosomes after Top2 inactivation, known to lead to a buildup of unresolved SCIs. This suggests that cohesin can influence the chromosomal association of Smc5/6 via its role in SCI protection. Cohesin is known to hold sister chromatid together until segregation occurs, and our results show that cohesin also controls Smc5/6, which is found to associate to linked chromatids specifically.
How could these nanomachines arise by natural means, in a gradual stepwise manner? Unless someone can demonstrate a series of small steps to climb mount improbable (as Richard Dawkins calls the challenge of evolving complex, information-rich, functional biological structures), this is wishful thinking. The mountain is not a series of small steps, but a sheer cliff with slippery vertical walls. And why would a mindless molecule even want to go climb uphill against its natural inclinations? The discoveries in biochemistry are making evolution increasingly untenable. Here we see highly complex molecules, made up of building blocks (amino acids) arranged in precise sequences to build functioning machines. The complexity is mind-boggling, and it exists all the way down in the very simplest single-celled life forms, with no precursors. Without these machines, the cell could not divide. Proposing intelligent design is not an argument of ignorance. We know that intelligent minds are capable of projecting complex machines where ideas of problem solutions are required. Intelligent minds are able to store large quantities of information into small spaces, computer chips are a good example. As a conclusion, Intelligent design constitutes the best, most causally adequate, explanation for the information in the cell.
1) http://genesdev.cshlp.org/content/26/15/1659.full
2) http://www.sciencedirect.com/science/article/pii/S0960982212012080
3) http://www.eurekalert.org/pub_releases/2006-07/ki-apc070306.php
4) http://www.ncbi.nlm.nih.gov/pubmed/21467147
5) http://www.ncbi.nlm.nih.gov/pmc/articles/PMC4199498/
6) http://crev.info/2004/07/spaghetti_in_a_basketball_how_the_cell_packs_dna_for_controlled_access/
7) http://creationsafaris.com/crev0802.htm
8 )https://en.wikipedia.org/wiki/SMC_protein
9) http://www.sciencedirect.com/science/article/pii/S0960982202010242
https://reasonandscience.catsboard.com/t2086-chromosome-condensation-amazing-evidence-of-design
Imagine trying to stuff about 10,000 miles of spaghetti inside a basketball. Then, if that was not difficult enough, attempt to find a unique one-inch segment of pasta from the middle of this mess, or try to duplicate, untangle and separate individual strings to opposite ends. This simple analogy illustrates some of the daunting tasks associated with the transcription, repair and replication of the nearly 2 meters of DNA that is packaged into the confines of a tiny eukaryotic nucleus. The solution to each of these problems lies in the assembly of the eukaryotic genome into chromatin, a structural polymer that not only solves the basic packaging problem but also provides a dynamic platform that controls all DNA-mediated processes within the nucleus.
Wrapping of DNA around a nucleosome core compacts the DNA length about sevenfold. The overall compaction in a chromosome, however, is greater than 10,000-fold—ample evidence for even higher orders of structural organization. Nucleosome cores are further organized into a structure called the 30 nm fiber. This packing requires one molecule of histone H1 per nucleosome core. The 30 nm fiber—a second level of chromatin organization—provides an approximately 100-fold compaction of the DNA. The next level of higher organization depends on nuclear scaffold proteins. The scaffold-associated regions are separated by loops of DNA. The DNA in a loop may contain a set of related genes. In Drosophila, for example, complete sets of histone-coding genes seem to cluster together in loops that are bounded by scaffold attachment sites. Additional layers of organization in eukaryotic chromosomes, each dramatically enhancing the degree of compaction.
Packing ratio - the length of DNA divided by the length into which it is packaged
The shortest human chromosome contains 4.6 x 107 bp of DNA (about 10 times the genome size of E. coli). This is equivalent to 14,000 µm of extended DNA, or about 2 meters. In its most condensed state during mitosis, the chromosome is about 2 µm long. This gives a packing ratio of 7000 (14,000/2).
To achieve the overall packing ratio, DNA is not packaged directly into the final structure of chromatin. Instead, it contains several hierarchies of organization.
The first level of packing is achieved by the winding of DNA around a protein core to produce a "bead-like" structure called a nucleosome. This gives a packing ratio of about 6. This structure is invariant in both the euchromatin and heterochromatin of all chromosomes.
The second level of packing is the coiling of beads in a helical structure called the 30 nm fiber that is found in both interphase chromatin and mitotic chromosomes. This structure increases the packing ratio to about 40.
The final packaging occurs when the fiber is organized in loops, scaffolds, and domains that give a final packing ratio of about 1000 in interphase chromosomes and about 7,000 in mitotic chromosomes.
That's an amazing change, from a ratio of 6 to 7.000 !!
Evolution, or design ??
Imagine trying to stuff about 10,000 miles of spaghetti inside a basketball. Then, if that was not difficult enough, attempt to find a unique one inch segment of pasta from the middle of this mess, or try to duplicate, untangle and separate individual strings to opposite ends. This simple analogy illustrates some of the daunting tasks associated with the transcription, repair and replication of the nearly 2 meters of DNA that is packaged into the confines of a tiny eukaryotic nucleus. The solution to each of these problems lies in the assembly of the eukaryotic genome into chromatin, a structural polymer that not only solves the basic packaging problem, but also provides a dynamic platform that controls all DNA-mediated processes within the nucleus. 6
Wrapping of DNA around a nucleosome core compacts the DNA length about sevenfold. The overall compaction in a chromosome, however, is greater than 10,000-fold—ample evidence for even higher orders of structural organization. In chromosomes isolated by very gentle methods, nucleosome cores seem to be organized into a structure called the 30 nm fiber.

The 30 nm fiber, a higher-order organization of nucleosomes.
(a) Schematic illustration of the probable structure of the fiber, showing nucleosome packing.
(b) Electron micrograph.
This packing requires one molecule of histone H1 per nucleosome core. Organization into 30 nm fibers does not extend over the entire chromosome but is punctuated by regions bound by sequence-specific (nonhistone) DNA-binding proteins. The 30 nm structure also seems to depend on the transcriptional activity of the particular region of DNA. Regions in which genes are being transcribed are apparently in a less-ordered state that contains little if any, histone H1.
The histone modifications do not disappear at cell division or during meiosis, and thus they become part of the information transmitted from one generation to the next in all eukaryotic organisms.
The 30 nm fiber—a second level of chromatin organization—provides an approximately 100-fold compaction of the DNA. Certain regions of DNA seem to associate with a nuclear scaffold. The scaffold-associated regions are separated by loops of DNA. The DNA in a loop may contain a set of related genes. In Drosophila, for example, complete sets of histone-coding genes seem to cluster together in loops that are bounded by scaffold attachment sites.

Loops of chromosomal DNA attached to a nuclear scaffold.
The DNA in the loops is packaged as 30 nm fibers, so the loops are the next level of organization. Loops often contain groups of genes with related functions. Complete sets of histone-coding genes, as shown in this schematic illustration, seem to be clustered in loops of this kind. Unlike most genes, histone genes occur in multiple copies in many eukaryotic genomes.
The scaffold itself may contain several proteins, notably large amounts of histone H1 (located in the interior of the fiber) and topoisomerase II. The presence of topoisomerase II further emphasizes the relationship between DNA underwinding and chromatin structure. Topoisomerase II is so important to the maintenance of chromatin structure that inhibitors of this enzyme can kill rapidly dividing cells. Evidence exists for additional layers of organization in eukaryotic chromosomes, each dramatically enhancing the degree of compaction.

Compaction of DNA in a eukaryotic chromosome.
This model shows the levels of organization that could provide the observed degree of DNA compaction in the chromosomes of eukaryotes. The levels take the form of coils upon coils. In cells, the higher-order structures (above
the 30 nm fibers) are unlikely to be as uniform as depicted here.
DNA compaction in eukaryotic chromosomes is likely to involve coils upon coils upon coils . . .
Every second, the cells constituting our bodies are replaced through cell division.An adult human consists of about 50,000 billion cells, 1% of which die and are replaced by cell division every day. In order to ensure cell survival and controlled growth of these new cells, the genetic information, stored in DNA molecules, must first be correctly copied and then accurately distributed during cell division. Moreover, to fully ascertain that the new cells will contain the same genetic information as the parental cells, any damage to the DNA, which is organised into several chromosomes, must be repaired. 3
Quite a bit is known about two of these complexes. One of them, cohesin, keeps the DNA copies together such that they do not separate too early; while the other, condensin, makes the chromosomes more compact, making the separation easier.
Prophase
During the first stage of mitosis, that of prophase, the duplicated chromosomes are prepared for segregation and the mitotic machinery is assembled.
Formation of the Mitotic Chromosome
The nucleus of an interphase cell contains tremendous lengths of chromatin fibers. The extended state of interphase chromatin is ideally suited for the processes of transcription and replication but not for segregation into two daughter cells. Before segregating its chromosomes, a cell converts them into much shorter, thicker structures by a remarkable process of chromosome compaction (or chromosome condensation), which occurs during early prophase

Research on chromosome compaction has focused on an abundant multiprotein complex called condensin.
Packing ratio - the length of DNA divided by the length into which it is packaged
The shortest human chromosome contains 4.6 x 107 bp of DNA (about 10 times the genome size of E. coli). This is equivalent to 14,000 µm of extended DNA, or about 2 meters. In its most condensed state during mitosis, the chromosome is about 2 µm long. This gives a packing ratio of 7000 (14,000/2).
To achieve the overall packing ratio, DNA is not packaged directly into the final structure of chromatin. Instead, it contains several hierarchies of organization.
The first level of packing is achieved by the winding of DNA around a protein core to produce a "bead-like" structure called a nucleosome. This gives a packing ratio of about 6. This structure is invariant in both the euchromatin and heterochromatin of all chromosomes.
The second level of packing is the coiling of beads in a helical structure called the 30 nm fiber that is found in both interphase chromatin and mitotic chromosomes. This structure increases the packing ratio to about 40.
The final packaging occurs when the fiber is organized in loops, scaffolds, and domains that give a final packing ratio of about 1000 in interphase chromosomes and about 7,000 in mitotic chromosomes.
That's an amazing change, from a ratio of 6 to 7.000 !!
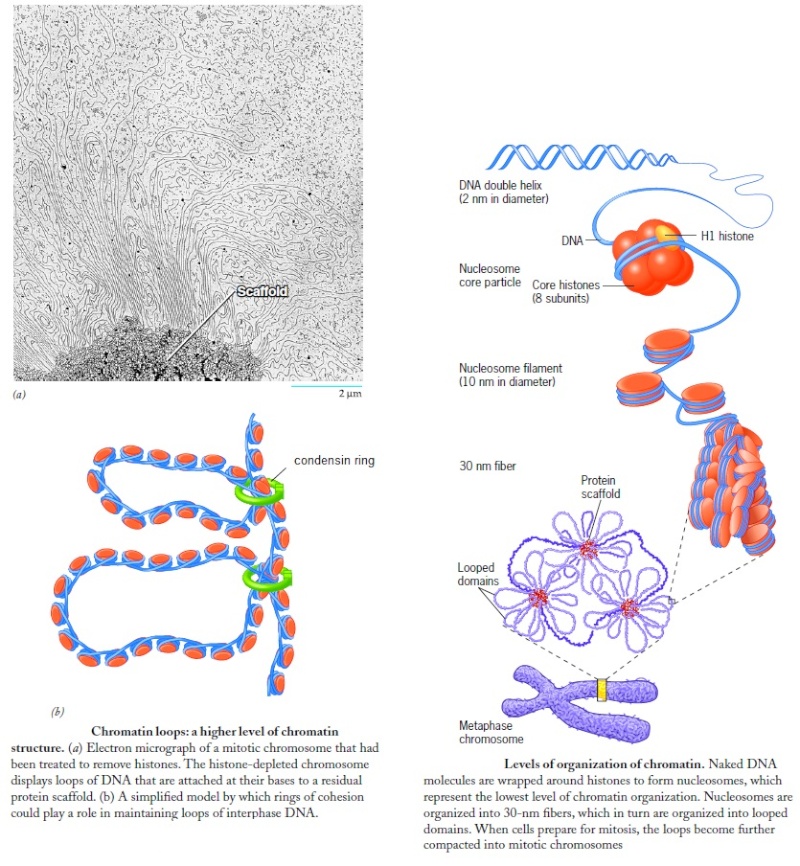
Squeezing DNA Into A Small Space
To fit 2 meters of DNA into a tiny nucleus is a monumental engineering feat. DNA is highly compacted yet has to be instantly available to rapidly make proteins in neurons with a momentary change of thought. This regulation is different in each type of cell. . It has been known for some time that the shape of proteins determines their function and the folding is very complex involving four levels of folding . Now it appears that the shape of the chromatin, also, determines function, with new secondary and tertiary structures discovered. 23
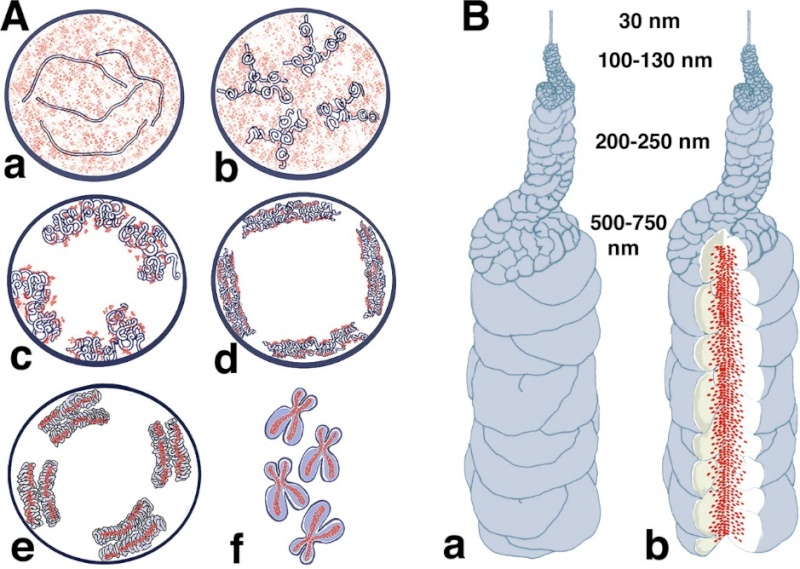
Models for chromosome condensation.
(A) Stages of condensation. Changes in large-scale chromatin folding (blue) versus SMC2 distribution (red) from early S (a), G2 (b), early prophase (c), middle prophase (d), late prophase (e), and metaphase (f). See text for details.
(B) “Hierarchical folding, axial glue” model of metaphase chromosome structure. (a) 30-nm fiber folds into 100–130-nm chromonema fiber, which folds into 200–250-nm middle prophase chromatid, which folds into 500–750-nm metaphase chromatid. Only one chromatid is shown here. (b) Axial condensin distribution (green) occupies approximately one-third of the chromatid diameter, acting as cross-linking “glue” to stabilize metaphase chromosome.
Condensins: universal organizers of chromosomes with diverse functions
Condensins are multisubunit protein complexes that play a fundamental role in the structural and functional organization of chromosomes in the three domains of life. It is a molecular machine that helps to condense and package chromosomes for cell replication. It is a five-subunit complex and is “the key molecular machine of chromosome condensation. 24
Condensin produces “super coils” of DNA, one of many steps in packing the delicate DNA strands into a hierarchy of coils that results in a densely-packed chromosome. “It is not entirely clear how the DNA is held in this supercoiled state,” they say, “but several studies suggest that the V-shaped arms of the condensin complex may loop and clamp the DNA in place.” This clamping is “rapid and reversible.” Scientists watching the process in both bacteria and humans are “showing that both vertebrate and bacterial condensins drive DNA compaction in an ATP-dependent fashion with a surprising level of co-operativity that was not fully appreciated.” The condensin molecules work as a team; if not enough condensin is around, nothing happens. These authors point out also that condensin is just one of many enzymes involved in chromosome formation. Think about how remarkable it is that during each cell division, the chromosomes are structured so reliably that they can be labeled and numbered under the microscope. “Our own proteomic analysis,” they claim, “has identified over 350 chromosome-associated proteins, so there is clearly more work to be done.” 6
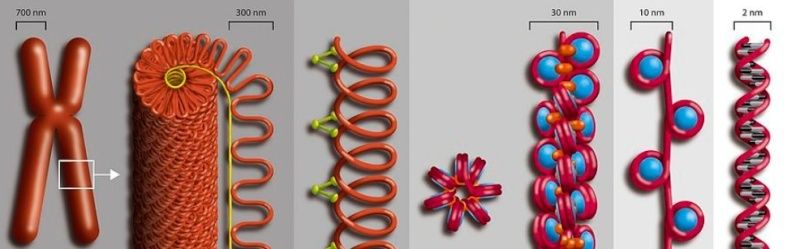
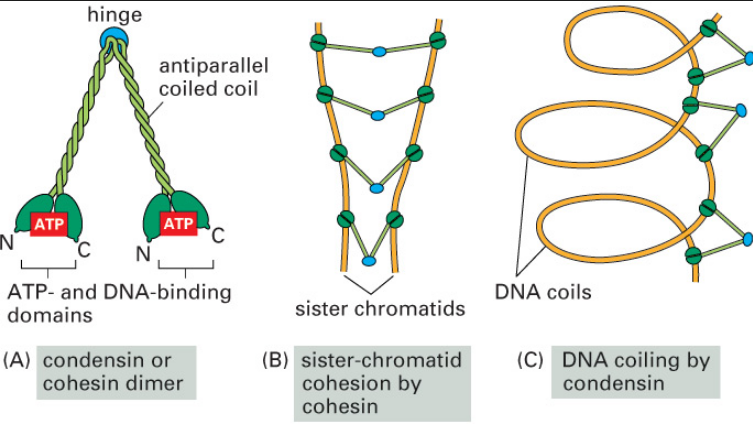
SMC proteins
SMC proteins represent a large family of ATPases that participate in many aspects of higher-order chromosome organization and dynamics. SMC stands for Structural Maintenance of Chromosomes.8
Eukaryotic SMCs
Eukaryotes have at least six SMC proteins in individual organisms, and they form three distinct heterodimers with specialized functions: A pair of SMC1 and SMC3 constitutes the core subunits of the cohesin complexes involved in sister chromatid cohesion. Likewise, a pair of SMC2 and SMC4 acts as the core of the condensin complexes implicated in chromosome condensation. A dimer composed of SMC5 and SMC6 functions as part of a yet-to-be-named complex implicated in DNA repair and checkpoint responses.
SMC proteins are conserved from bacteria to humans.
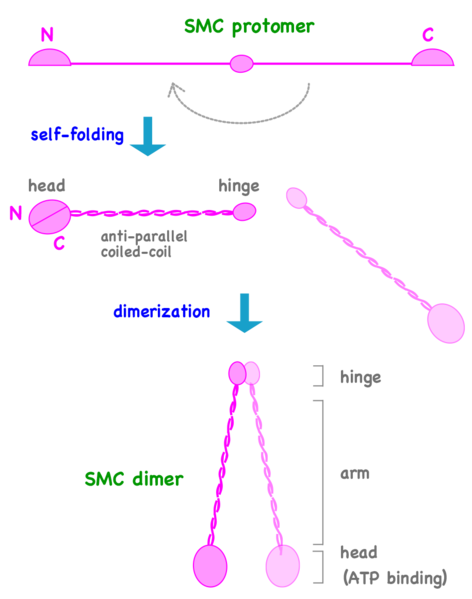
Can We Live Without SMCs? No!
The Structural Maintenance of Chromosomes (SMC) proteins are a family of chromosomal ATPases highly conserved among the three phyla of life. ... What do SMCs look like? The SMC proteins are large polypeptides, each spanning 1000-1500 amino acids. They form dimers in which two anti-parallel coiled-coil arms are connected by a flexible hinge. ... The distal end of each arm constitutes an ATP-binding domain.7
This family of proteins look like tweezers that can grab DNA and keep it under control during critical processes in the cell. They are important in holding sister chromatids together and separating them during cell division. Some are also “implicated in DNA repair and checkpoint responses.” They are also essential for the proper separation of chromosomes during gamete formation during meiotic cell division. So how do these miniature grappling hooks do all this? How do SMCs work? That is the million-dollar question in the field. Of particular interest is to understand how the two-armed structure - which is approximately 100 nm long when it’s open! - captures DNA, and how these interactions are modulated by ATP binding and hydrolysis. Condensin is able to introduce positive supercoils into DNA by using the energy of ATP hydrolysis.
Can we live without SMCs? No! Loss of any single SMC protein in budding yeast is lethal.
The short article by Gillespie and Hirano (Cold Spring Harbor Laboratories, NY) contains a diagram of what two sample SMC proteins look like. The SMC “superfamily” work in complexes with other molecules to accomplish these vital tasks. 9
Condensins are multisubunit protein complexes that play a fundamental role in the structural and functional organization of chromosomes in the three domains of life. 1
The chromosomal condensin complex is a major molecular effector of chromosome condensation and segregation in diverse organisms ranging from bacteria to humans. Condensin is a large, evolutionarily conserved, multisubunit protein assembly composed of dimers of the structural maintenance of chromosomes (SMC) family of ATPases, clasped into topologically closed rings by accessory subunits. Condensin binds to DNA dynamically, in a poorly understood cycle of ATP-modulated conformational changes, and exhibits the ability to positively supercoil DNA. During mitosis, condensin is phosphorylated by the cyclin-dependent kinase (CDK), Polo and Aurora B kinases in a manner that correlates with changes in its localisation, dynamics and supercoiling activity.
Condensin helps configure duplicated chromosomes for separation
At the end of S phase, the immensely long DNA molecules of the sister chromatids are tangled in a mass of partially catenated DNA and proteins. Any attempt to pull the sisters apart in this state would undoubtedly lead to breaks in the chromosomes. To avoid this disaster, the cell devotes a great deal of energy in early mitosis to gradually reorganizing the sister chromatids into relatively short, distinct structures that can be pulled apart more easily in anaphase. These chromosomal changes involve two processes: chromosome condensation, in which the chromatids are dramatically compacted; and sister-chromatid resolution, whereby the two sisters are resolved into distinct, separable units

Resolution results from the decatenation of the sister DNAs, accompanied by the partial removal of cohesin molecules along the chromosome arms.
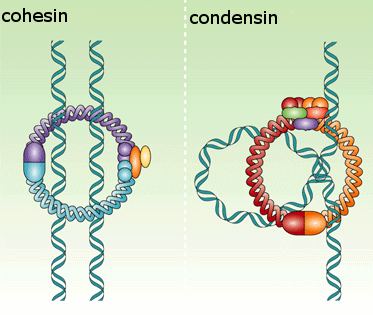
As a result, when the cell reaches metaphase, the sister chromatids appear in the microscope as compact, rod-like structures that are joined tightly at their centromeric regions and only loosely along their arms.The condensation and resolution of sister chromatids depends, at least in part, on a five-subunit protein complex called condensin. Condensin structure is related to that of the cohesin complex that holds sister chromatids together
It contains two SMC subunits like those of cohesin, plus three non-SMC subunits
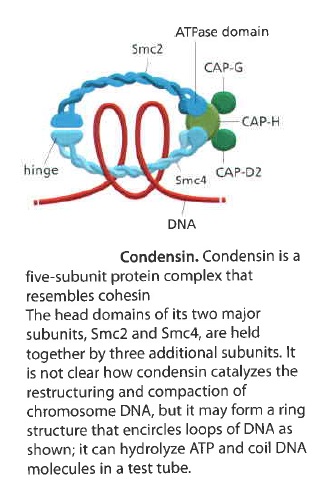
Condensin may form a ring-like structure that somehow uses the energy provided byATP hydrolysis to promote the compaction and resolution of sister chromatids. Condensin is able to change the coiling of DNA molecules in a test tube, and this coiling activity is thought to be important for chromosome condensation during mitosis. Interestingly, phosphorylation of condensin subunits by M-Cdk stimulates this coiling activity, providing one mechanism by which M-Cdk may promote chromosome restructuring in early mitosis.
There is third, less well understood, protein complex, known as the Smc5/6 complex. 3 This protein complex was found to bind to locations on the DNA strand that the researchers had artificially damaged, suggesting that it is directly involved in the repair process. Moreover, the Smc5/6 complex also seems to be required for the disentanglement of undamaged chromosomes before cell division. If these tangles, which are a natural consequence of the DNA copying process, are left unresolved the chromosomes cannot be separated and sent to the two nascent daughter cells. Like in the repair process, the Smc5/6 complex appears to resolve these intertwines by direct interaction with the DNA molecules, but this process is differently regulated as compared to the function in repair.
"Evidence points to that the Smc5/6 complex work in two different pathways, one needed for repair and the other for untangling.
The Smc5/6 complex: more than repair?
Through its functions in chromosome replication, segregation, and repair, the Smc5/6 complex has a central role in the maintenance of genome stability. The complex is part of the family of structural maintenance of chromosome protein complexes that also includes cohesin and condensin. Mutations in any of these complexes disrupt chromosome segregation and render cells hypersensitive to different types of DNA damage. The chromosome mis-segregation phenotypes in cohesin and condensin mutants can be attributed to their functions in sister chromatid cohesion and chromosome condensation, respectively. Cohesin-dependent chromatid cohesion is also needed for DNA double-strand break repair, whereas condensin is required for repair of single-strand breaks. How Smc5/6 promotes chromosome stability is largely unknown. Accumulating data suggest that it prevents accumulation of aberrant DNA links between sister chromatids created during repair by homologous recombination. A long-standing idea is that it also has a role in the maintenance of nondamaged chromosomes. Here, we present an overview of the current knowledge of Smc5/6 and discuss a possible non-repair role of the complex. 4
The Chromosomal Association of the Smc5/6 Complex Depends on Cohesion and Predicts the Level of Sister Chromatid Entanglement 5
The cohesin complex, which is essential for sister chromatid cohesion and chromosome segregation, also inhibits resolution of sister chromatid intertwinings (SCIs) by the topoisomerase Top2. The cohesin-related Smc5/6 complex (Smc5/6) instead accumulates on chromosomes after Top2 inactivation, known to lead to a buildup of unresolved SCIs. This suggests that cohesin can influence the chromosomal association of Smc5/6 via its role in SCI protection. Cohesin is known to hold sister chromatid together until segregation occurs, and our results show that cohesin also controls Smc5/6, which is found to associate to linked chromatids specifically.
How could these nanomachines arise by natural means, in a gradual stepwise manner? Unless someone can demonstrate a series of small steps to climb mount improbable (as Richard Dawkins calls the challenge of evolving complex, information-rich, functional biological structures), this is wishful thinking. The mountain is not a series of small steps, but a sheer cliff with slippery vertical walls. And why would a mindless molecule even want to go climb uphill against its natural inclinations? The discoveries in biochemistry are making evolution increasingly untenable. Here we see highly complex molecules, made up of building blocks (amino acids) arranged in precise sequences to build functioning machines. The complexity is mind-boggling, and it exists all the way down in the very simplest single-celled life forms, with no precursors. Without these machines, the cell could not divide. Proposing intelligent design is not an argument of ignorance. We know that intelligent minds are capable of projecting complex machines where ideas of problem solutions are required. Intelligent minds are able to store large quantities of information into small spaces, computer chips are a good example. As a conclusion, Intelligent design constitutes the best, most causally adequate, explanation for the information in the cell.
1) http://genesdev.cshlp.org/content/26/15/1659.full
2) http://www.sciencedirect.com/science/article/pii/S0960982212012080
3) http://www.eurekalert.org/pub_releases/2006-07/ki-apc070306.php
4) http://www.ncbi.nlm.nih.gov/pubmed/21467147
5) http://www.ncbi.nlm.nih.gov/pmc/articles/PMC4199498/
6) http://crev.info/2004/07/spaghetti_in_a_basketball_how_the_cell_packs_dna_for_controlled_access/
7) http://creationsafaris.com/crev0802.htm
8 )https://en.wikipedia.org/wiki/SMC_protein
9) http://www.sciencedirect.com/science/article/pii/S0960982202010242
Last edited by Admin on Tue Oct 09, 2018 4:22 am; edited 29 times in total