Microfilaments
Microfilaments are much thinner than microtubules. They have a diameter of about 7 nm, which makes them the smallest of the major cytoskeletal components. Microfilaments are best known for their role in the contractile fibrils of muscle cells. However, microfilaments are involved in a variety of other cellular phenomena as well. They can form connections with the plasma membrane and thereby influence locomotion, amoeboid movement, and cytoplasmic streaming. Microfilaments also produce the cleavage furrow that divides the cytoplasm of an animal cell after the two sets of chromosomes have been
separated by the mitotic spindle fibers. In addition, microfilaments contribute importantly to the development and maintenance of cell shape. Microfilaments are polymers of the protein actin. Actin is synthesized as a monomer called G-actin (G for globular). G-actin monomers polymerize into long strands of F-actin (F for filamentous), with each strand about 4 nm wide. Each microfilament consists of a chain of actin monomers that are assembled into a filament with a helical appearance and a diameter of about 7 nm. Like microtubules, microfilaments show polarity; all of the subunits are oriented in the same direction.
This polarity influences the direction of microfilament elongation; assembly usually proceeds more readily at one end of the growing microfilament, whereas disassembly is favored at the other end.
Cytoskeletal Filaments Adapt to Form Dynamic or Stable Structures
Cytoskeletal systems are dynamic and adaptable, organized more like ant trails than interstate highways. A single trail of ants may persist for many hours, extending from the ant nest to a delectable picnic site, but the individual ants within the trail are anything but static. If the ant scouts find a new and better source of food, or if the picnickers clean up and leave, the dynamic structure adapts with astonishing rapidity. In a similar way, large-scale cytoskeletal structures can change or persist, according to need, lasting for lengths of time ranging from less than a minute up to the cell’s lifetime. But the individual macromolecular components that make up these structures are in a constant state of flux. Thus, like the alteration of an ant trail, a structural rearrangement in a cell requires little extra energy when conditions change. Regulation of the dynamic behavior and assembly of cytoskeletal filaments allows eukaryotic cells to build an enormous range of structures from the three basic filament systems.


The micrographs above illustrate some of these structures. Actin filaments underlie the plasma membrane of animal cells, providing strength and shape to its thin lipid bilayer. They also form many types of cell-surface projections. Some of these are dynamic structures, such as the lamellipodia and filopodia that cells use to explore territory and move around. More stable arrays allow cells to brace themselves against an underlying substratum and enable muscle to contract. The regular bundles of stereocilia on the surface
of hair cells in the inner ear contain stable bundles of actin filaments that tilt as rigid rods in response to sound, and similarly organized microvilli on the surface of intestinal epithelial cells vastly increase the apical cell-surface area to enhance nutrient absorption. In plants, actin filaments drive the rapid streaming of cytoplasm inside cells.Microtubules, which are frequently found in a cytoplasmic array that extends to the cell periphery, can quickly rearrange themselves to form a bipolar mitotic spindle during cell division. They can also form cilia, which function as motile whips or sensory devices on the surface of the cell, or tightly aligned bundles that serve as tracks for the transport of materials down long neuronal axons. In plant cells, organized arrays of microtubules help to direct the pattern of cell wall synthesis, and in many protozoans they form the framework upon which the entire cell is built. Intermediate filaments line the inner face of the nuclear envelope, forming a protective cage for the cell’s DNA; in the cytosol, they are twisted into strong cables that can hold epithelial cell sheets together or help nerve cells to extend long and robust axons, and they allow us to form tough appendages such as hair and fingernails. An important and dramatic example of rapid reorganization of the cytoskeleton occurs during cell division, as shown below for a fibroblast growing in a tissue-culture dish.:

After the chromosomes have replicated, the interphase microtubule array that spreads throughout the cytoplasm is reconfigured into the bipolar mitotic spindle, which segregates the two copies of each chromosome into daughter nuclei. At the same time, the specialized actin structures that enable the fibroblast to crawl across the surface of the dish rearrange so that the cell stops moving and assumes a more spherical shape. Actin and its associated motor protein myosin then form a belt around the middle of the cell, the contractile ring, which constricts like a tiny muscle to pinch the cell in two. When division is complete, the cytoskeletons of the two daughter fibroblasts reassemble into theirinterphase structures to convert the two rounded-up daughter cells into smaller versions of the flattened, crawling mother cell. Many cells require rapid cytoskeletal rearrangements for their normal functioning during interphase as well.
The Cytoskeleton Determines Cellular Organization and Polarity
In cells that have achieved a stable, differentiated morphology—such as mature neurons or epithelial cells—the dynamic elements of the cytoskeleton must also provide stable, large-scale structures for cellular organization. On specialized epithelial cells that line organs such as the intestine and the lung, cytoskeletal-based cell-surface protrusions including microvilli and cilia are able to maintain a constant location, length, and diameter over the entire lifetime of the cell. For the actin bundles at the cores of microvilli on intestinal epithelial cells, this is only a few days. But the actin bundles at the cores of stereocilia on the hair cells of the inner ear must maintain their stable organization for the entire lifetime of the animal, since these cells do not turn over. Nonetheless, the individual actin filaments remain strikingly dynamic and are continuously remodeled and replaced every 48 hours, even within stable cell-surface structures that persist for decades. Besides forming stable, specialized cell-surface protrusions, the cytoskeleton is also responsible for large-scale cellular polarity, enabling cells to tell the difference between top and bottom, or front and back. The large-scale polarity information conveyed by cytoskeletal organization is often maintained over the lifetime of the cell. Polarized epithelial cells use organized arrays of microtubules, actin filaments, and intermediate filaments to maintain the critical differences between the apical surface and the basolateral surface. They also must maintain strong adhesive contacts with one another to enable this single layer of cells to serve as an effective physical barrier

Filaments Assemble from Protein Subunits That Impart Specific Physical and Dynamic Properties
Cytoskeletal filaments can reach from one end of the cell to the other, spanning tens or even hundreds of micrometers. Yet the individual protein molecules that form the filaments are only a few nanometers in size. The cell builds the filaments by assembling large numbers of the small subunits, like building a skyscraper out of bricks. Because these subunits are small, they can diffuse rapidly in the cytosol, whereas the assembled filaments cannot. In this way, cells can undergo rapid structural reorganizations, disassembling filaments at one site and reassembling them at another site far away. Actin filaments and microtubules are built from subunits that are compact and globular—actin subunits for actin filaments and tubulin subunits for microtubules— whereas intermediate filaments are made up of smaller subunits that are themselves elongated and fibrous. All three major types of cytoskeletal filaments form as helical assemblies of subunits that self-associate, using a combination of end-to-end and side-to-side protein contacts. Differences in the structures of the subunits and the strengths of the attractive forces between them produce important differences in the stability and mechanical properties of each type of filament. Whereas covalent linkages between their subunits hold together the backbones of many biological polymers—including DNA, RNA, and proteins—it is weak noncovalent interactions that hold together the three types of cytoskeletal polymers. Consequently, their assembly and disassembly can occur rapidly, without covalent bonds being formed or broken.
The subunits of actin filaments and microtubules are asymmetrical and bind to one another head-to-tail so that they all point in one direction. This subunit polarity gives the filaments structural polarity along their length, and makes the two ends of each polymer behave differently. In addition, actin and tubulin subunits are both enzymes that catalyze the hydrolysis of a nucleoside triphosphate— ATP and GTP, respectively. The energy derived from nucleotide hydrolysis enables the filaments to remodel rapidly. By controlling when and where actin and microtubules assemble, the cell harnesses the polar and dynamic properties of these filaments to generate force in a specific direction, to move theleading edge of a migrating cell forward, for example, or to pull chromosomes apart during cell division. In contrast, the subunits of intermediate filaments are symmetrical, and thus do not form polarized filaments with two different ends. Intermediate filament subunits also do not catalyze the hydrolysis of nucleotides. Nevertheless, intermediate filaments can be disassembled rapidly when required. In mitosis , for example, kinases phosphorylate the subunits, leading to their dissociation. Cytoskeletal filaments in living cells are not built by simply stringing subunits together in single file. A thousand tubulin subunits lined up end-to-end, for example, would span the diameter of a small eukaryotic cell, but a filament formed in this way would lack the strength to avoid breakage by ambient thermal energy, unless each subunit in the filament was bound extremely tightly to its two neighbors. Such tight binding would limit the rate at which the filaments could disassemble, making the cytoskeleton a static and less useful structure. To provide both strength and adaptability, microtubules are built of 13 protofilaments—linear strings of subunits joined end-to-end—that associate with one another laterally to form a hollow cylinder. The addition or loss of a subunit at the end of one protofilament makes or breaks a small number of bonds. In contrast, loss of a subunit from the middle of the filament requires breaking many more bonds, while breaking it in two requires breaking bonds in multiple protofilaments all at the same time

The greater energy required to break multiple noncovalent bonds simultaneously allows microtubules to resist thermal breakage, while allowing rapid subunit addition and loss at the filament ends. Helical actin filaments are much thinner and therefore require much less energy to break. However, multiple actin filaments are often bundled together inside cells, providing mechanical strength, while allowing dynamic behavior of filament ends. As with other specific protein–protein interactions, many hydrophobic interactions and noncovalent bonds hold the subunits in a cytoskeletal filament togethe . The locations and types of subunit–subunit contacts differ for the different filaments. Intermediate filaments, for example, assemble by forming strong lateral contacts between α-helical coiled-coils, which extend over most of the length of each elongated fibrous subunit. Because the individual subunits are staggered in the filament, intermediate filaments form strong, ropelike structures that tolerate stretching and bending to a greater extent than do either actin filaments or microtubules
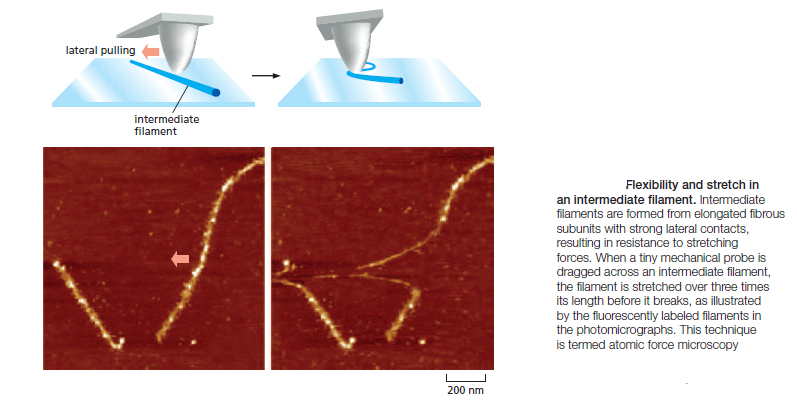
Accessory Proteins and Motors Regulate Cytoskeletal Filaments
The cell regulates the length and stability of its cytoskeletal filaments, as well as their number and geometry. It does so largely by regulating their attachments to one another and to other components of the cell, so that the filaments can form a wide variety of higher-order structures. Direct covalent modification of the filament subunits regulates some filament properties, but most of the regulation is performed by hundreds of accessory proteins that determine the spatial distribution and the dynamic behavior of the filaments, converting information received through signaling pathways into cytoskeletal action. These accessory proteins bind to the filaments or their subunits to determine the sites of assembly of new filaments, to regulate the partitioning of polymer proteins between filament and subunit forms, to change the kinetics of filament assembly and disassembly, to harness energy to generate force, and to link filaments to one another or to other cell structures such as organelles and the plasma membrane. In these processes, the accessory proteins bring cytoskeletal structure under the control of extracellular and intracellular signals, including those that trigger the dramatic transformations of the cytoskeleton that occur during each cell cycle. Acting together, the accessory proteins enable a eukaryotic cell to maintain a highly organized but flexible internal structure and, in many cases, to move. Among the most fascinating proteins that associate with the cytoskeleton are the motor proteins. These proteins bind to a polarized cytoskeletal filament and
use the energy derived from repeated cycles of ATP hydrolysis to move along it. Dozens of different motor proteins coexist in every eukaryotic cell. They differ in the type of filament they bind to (either actin or microtubules), the direction in which they move along the filament, and the “cargo” they carry. Many motor proteins carry membrane-enclosed organelles—such as mitochondria, Golgi stacks, or secretory vesicles—to their appropriate locations in the cell. Other motor proteins cause cytoskeletal filaments to exert tension or to slide against each other, generating the force that drives such phenomena as muscle contraction, ciliary beating, and cell division. Cytoskeletal motor proteins that move unidirectionally along an oriented polymer track are reminiscent of some other proteins and protein complexes , such as DNA and RNA polymerases, helicases, and
ribosomes. All of these proteins have the ability to use chemical energy to propel themselves along a linear track, with the direction of sliding dependent on the structural polarity of the track. All of them generate motion by coupling nucleoside triphosphate hydrolysis to a large-scale conformational change
Bacterial Cell Organization and Division Depend on Homologs of Eukaryotic Cytoskeletal Proteins
While eukaryotic cells are typically large and morphologically complex, bacterial cells are usually only a few micrometers long and assume simple shapes such as spheres or rods. Bacteria also lack elaborate networks of intracellular membrane- enclosed organelles. Historically, biologists assumed that a cytoskeleton was not necessary in such simple cells. We now know, however, that bacteria contain homologs of all three of the eukaryotic cytoskeletal filaments. Furthermore, bacterial actins and tubulins are more diverse than their eukaryotic versions, both in the types of assemblies they form and in the functions they carry out. Nearly all bacteria and many archaea contain a homolog of tubulin called FtsZ,which can polymerize into filaments and assemble into a ring (called the Z-ring) at the site where the septum forms during cell division

The bacterial FtsZ protein, a tubulin homolog in prokaryotes.
(A) A band of FtsZ protein forms a ring in a dividing bacterial cell. This ring has been labeled by fusing the FtsZ protein to green fluorescent protein (GFP), which allows it to be observed in living E. coli cells with a fluorescence microscope.
(B) FtsZ filaments and circles, formed in vitro, as visualized using electron microscopy.
(C) Dividing chloroplasts (red) from a red alga also cleave using a protein ring made from FtsZ (yellow).
Although the Z-ring persists for many minutes, the individual filaments within it are highly dynamic, with an average filament half-life of about thirty seconds. As the bacterium
divides, the Z-ring becomes smaller until it has completely disassembled. FtsZ filaments in the Z-ring are thought to generate a bending force that drives the membrane invagination necessary to complete cell division. The Z-ring may also serve as a site for localization of enzymes required for building the septum between the two daughter cells.Many bacteria also contain homologs of actin. Two of these, MreB and Mbl, are found primarily in rod-shaped or spiral-shaped cells where they assemble to form dynamic patches that move circumferentially along the length of the cell
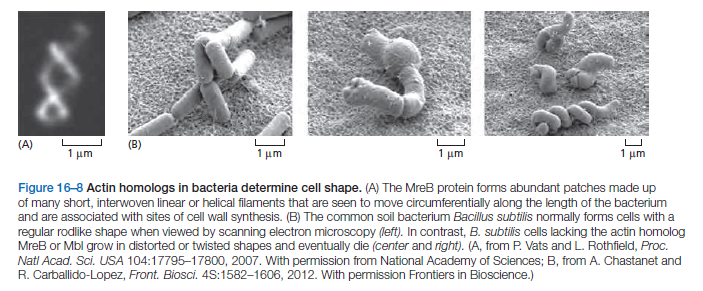
These proteins contribute to cell shape by serving as a scaffold to direct the synthesis of the peptidoglycan cell wall, in much the same way that microtubules help organize the synthesis of the cellulose cell wall in higher plant cells . As with FtsZ, MreB and Mbl filaments are highly dynamic, with half-lives of a few minutes, and nucleotide hydrolysis accompanies the polymerization process. Mutations disrupting MreB or Mbl expression cause extreme abnormalities in cell shape and defects in chromosome segregation (Figure
B above).
ACTIN AND ACTIN-BINDING PROTEINS
The actin cytoskeleton performs a wide range of functions in diverse cell types. Each actin subunit, sometimes called globular or G-actin, is a 375-amino-acid polypeptide carrying a tightly associated molecule of ATP or ADP

The structures of an actin monomer and actin filament.
(A) The actin monomer has a nucleotide (either ATP or ADP) bound in a deep cleft in the center of the molecule.
(B) Arrangement of monomers in a filament consisting of two protofilaments, held together by lateral contacts, which wind around each other as two parallel strands of a helix, with a twist repeating every 37 nm. All the subunits within the filament have the same orientation.
(C) Electron micrograph of negatively stained actin filament.
Actin is extraordinarily well conserved among eukaryotes. The amino acid sequences of actins from different eukaryotic species are usually about 90% identical. Small variations in actin amino acid sequence can cause significant functional differences: In vertebrates, for example, there are three isoforms of actin, termed α, β, and γ, that differ slightly in their amino acid sequences and have distinct functions. α-Actin is expressed only in muscle cells, while β- and γ-actins are found together in almost all non-muscle cells.
Actin Subunits Assemble Head-to-Tail to Create Flexible, Polar Filaments
Actin subunits assemble head-to-tail to form a tight, right-handed helix, forming a structure about 8 nm wide called filamentous or F-actin (Figure B and C above). Because the asymmetrical actin subunits of a filament all point in the same direction, filaments are polar and have structurally different ends: a slower-growing minus end and a faster-growing plus end. The minus end is also referred to as the “pointed end” and the plus end as the “barbed end,” because of the “arrowhead” appearance of the complex formed between actin filaments and the motor protein myosin

Within the filament, the subunits are positioned with their nucleotide-binding cleft directed toward the minus end. Individual actin filaments are quite flexible. The stiffness of a filament can be characterized by its persistence length, the minimum filament length at which random thermal fluctuations are likely to cause it to bend. The persistence length of an actin filament is only a few tens of micrometers. In a living cell, however, accessory proteins cross-link and bundle the filaments together, making largescale actin structures that are much more rigid than an individual actin filament
Nucleation Is the Rate-Limiting Step in the Formation of Actin Filaments
The regulation of actin filament formation is an important mechanism by which cells control their shape and movement. Small oligomers of actin subunits can assemble spontaneously, but they are unstable and disassemble readily because each monomer is bound to only one or two other monomers. For a new actin filament to form, subunits must assemble into an initial aggregate, or nucleus, that is stabilized by multiple subunit–subunit contacts and can then elongate rapidly by addition of more subunits. This process is called filament nucleation. Many features of actin nucleation and polymerization have been studied with purified actin in a test tube
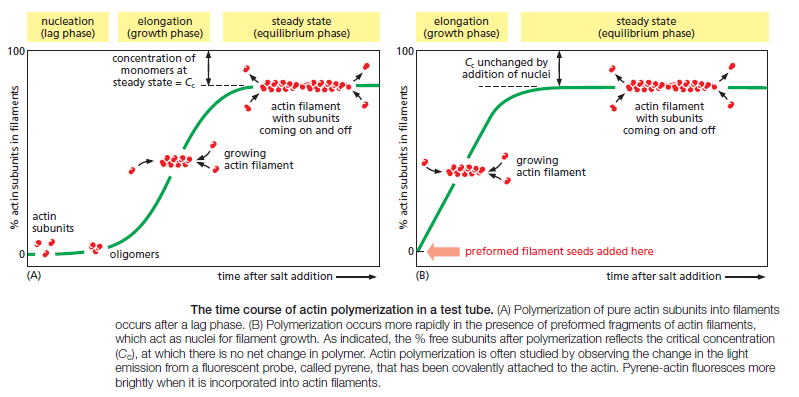
The instability of smaller actin aggregates creates a kinetic barrier to nucleation. When polymerization is initiated, this barrier results in a lag phase during which no filaments are observed. During this lag phase, however, a few of the small, unstable aggregates succeed in making the transition to a more stable form that resembles an actin filament. This leads to a phase of rapid filament elongation during which subunits are added quickly to the ends of the nucleated filaments (Figure A above). Finally, as the concentration
of actin monomers declines, the system approaches a steady state at which the rate of addition of new subunits to the filament ends exactly balances the rate of subunit dissociation. The concentration of free subunits left in solution at this point is called the critical concentration, Cc. The value of the critical concentration is equal to the rate constant for subunit loss divided by the rate constant for subunit addition—that is, Cc = koff/kon, which is equal to the dissociation constant, Kd, and the inverse of the equilibrium constant, K. In a test tube, the Cc for actin polymerization—that is, the free actin monomer concentration at which the fraction of actin in the polymer stops increasing—is about 0.2 μM. Inside the cell, the concentration of unpolymerized actin is much higher than this, and the cell has mechanisms to prevent most of its monomeric actin from assembling into filamentsr.
Question : how did these mechanisms emerge ? how did the function to assemble the right amount of actin filaments emerge ? How did the monomeric units emerge with the right fit and the right size and the right quantity ? trial and error ? had these filaments not have to be present right from the start, in order to get the right cell shape ?
The lag phase in filament growth is eliminated if preexisting seeds (such as fragments of actin filaments that have been chemically cross-linked) are added to the solution at the beginning of the polymerization reaction (Figure B above). The cell takes great advantage of this nucleation requirement: it uses special proteins to catalyze filament nucleation at specific sites, thereby determining the location at which new actin filaments are assembled.
Actin Filaments Have Two Distinct Ends That Grow at Different Rates
Due to the uniform orientation of asymmetric actin subunits in the filament, the structures at its two ends are different. This orientation makes the two ends of each polymer different in ways that have a profound effect on filament growth rates. The kinetic rate constants for actin subunit association and dissociation— kon and koff, respectively—are much greater at the plus end than the minus end. This can be seen when an excess of purified actin monomers is allowed to assemble onto polarity-marked filaments—the plusend of the filament elongates up to ten times faster . If filaments are rapidly diluted so that the free subunit concentration drops below the critical concentration, the plus end also
depolymerizes faster. It is important to note, however, that the two ends of an actin filament have the same net affinity for actin subunits, if all of the subunits are in the same nucleotide state. Addition of a subunit to either end of a filament of n subunits results in a filament of n + 1 subunits. Thus, the free-energy difference, and therefore the equilibrium constant (and the critical concentration), must be the same for addition of subunits at either end of the polymer. In this case, the ratio of the rate constants,
koff/kon, must be identical at the two ends, even though the absolute values of these rate constants are very different at each end. The cell takes advantage of actin filament dynamics and polarity to do mechanical work. Filament elongation proceeds spontaneously when the free-energy change (ΔG) for addition of the soluble subunit is less than zero. This is the case when the concentration of subunits in solution exceeds the critical concentration. A cell can couple an energetically unfavorable process to this spontaneous
process; thus, the cell can use free energy released during spontaneous filament polymerization to move an attached load. For example, by orienting the fast-growing plus ends of actin filaments toward its leading edge, a motile cell can push its plasma membrane forward.
ATP Hydrolysis Within Actin Filaments Leads to Treadmilling at Steady State
Thus far in our discussion of actin filament dynamics, we have ignored the critical fact that actin can catalyze the hydrolysis of the nucleoside triphosphate ATP. For free actin subunits, this hydrolysis proceeds very slowly; however, it is accelerated when the subunits are incorporated into filaments. Shortly after ATP hydrolysis occurs, the free phosphate group is released from each subunit, but the ADP remains trapped in the filament structure. Thus, two different types of filament structures can exist, one with the “T form” of the nucleotide bound (ATP), and one with the “D form” bound (ADP). When the nucleotide is hydrolyzed, much of the free energy released by cleavage of the phosphate–phosphate bond is stored in the polymer. This makes the free-energy change for dissociation of a subunit from the D-form polymer more negative than the free-energy change for dissociation of a subunit from the T-form polymer. Consequently, the ratio of koff/kon for the D-form polymer, which is numerically equal to its critical concentration [Cc(D)], is larger than the corresponding ratio for the T-form polymer. Thus, Cc(D) is greater than Cc(T). At certain concentrations of free subunits, D-form polymers will therefore shrink while T-form polymers grow.
In living cells, most soluble actin subunits are in the T form, as the free concentration of ATP is about tenfold higher than that of ADP. However, the longer the time that subunits have been in the actin filament, the more likely they are to have hydrolyzed their ATP. Whether the subunit at each end of a filament is in the T or the D form depends on the rate of this hydrolysis compared with the rate of subunit addition. If the concentration of actin monomers is greater than the critical concentration for both the T-form and D-form polymer, then subunits will add to the polymer at both ends before the nucleotides in the previously added subunits are hydrolyzed; as a result, the tips of the actin filament will remain in the T form. On the other hand, if the subunit concentration is less than the critical concentrations for both the T-form and D-form polymer, then hydrolysis may occur before the next subunit is added and both ends of the filament will be in the D form and will shrink. At intermediate concentrations of actin subunits, it is possible for the rate of subunit addition to be faster than nucleotide hydrolysis at the plus end, but slower than nucleotide hydrolysis at the minus end. In this case, the plus end of the filament remains in the T conformation, while the minus end adopts the D conformation. The filament then undergoes a net addition of subunits at the plus end, while simultaneously losing subunits from the minus end. This leads to the remarkable property of filament treadmilling

At a particular intermediate subunit concentration, the filament growth at the plus end exactly balances the filament shrinkage at the minus end. Under these conditions, the subunits cycle rapidly between the free and filamentous states

while the total length of the filament remains unchanged. This “steady-state treadmilling requires a constant consumption of energy in the form of ATP hydrolysis. Within a cell, actin behavior is regulated by numerous accessory proteins that bind actin monomers or filaments
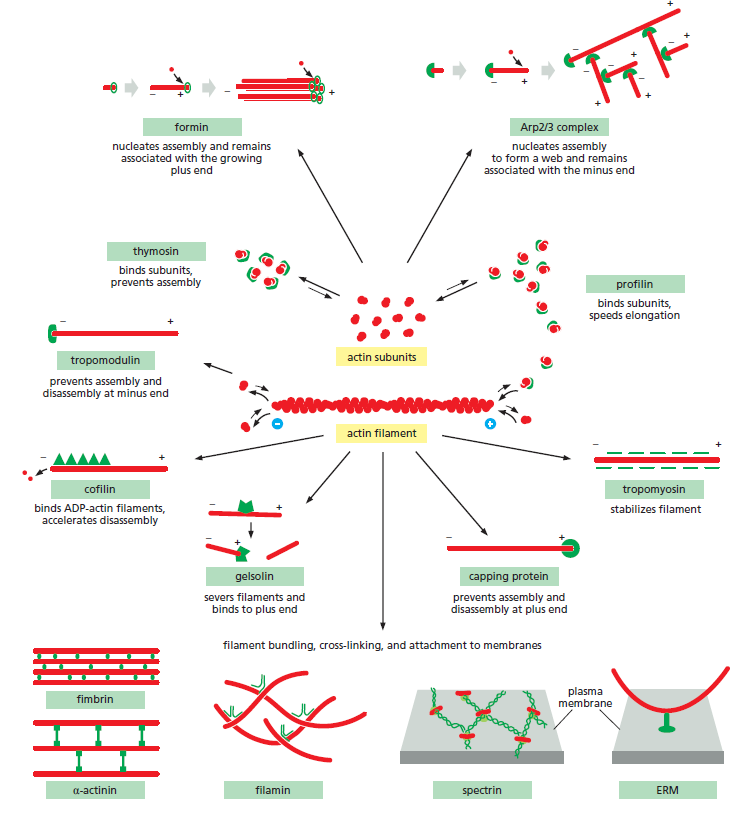
Some of the major accessory proteins of the actin cytoskeleton. Except for the myosin motor proteins, an example of each major type is shown. Each of these is discussed in the text. However, most cells contain more than a hundred different actin-binding proteins, and it is likely that there are important types of actin-associated proteins that are not yet recognized
Microfilaments are much thinner than microtubules. They have a diameter of about 7 nm, which makes them the smallest of the major cytoskeletal components. Microfilaments are best known for their role in the contractile fibrils of muscle cells. However, microfilaments are involved in a variety of other cellular phenomena as well. They can form connections with the plasma membrane and thereby influence locomotion, amoeboid movement, and cytoplasmic streaming. Microfilaments also produce the cleavage furrow that divides the cytoplasm of an animal cell after the two sets of chromosomes have been
separated by the mitotic spindle fibers. In addition, microfilaments contribute importantly to the development and maintenance of cell shape. Microfilaments are polymers of the protein actin. Actin is synthesized as a monomer called G-actin (G for globular). G-actin monomers polymerize into long strands of F-actin (F for filamentous), with each strand about 4 nm wide. Each microfilament consists of a chain of actin monomers that are assembled into a filament with a helical appearance and a diameter of about 7 nm. Like microtubules, microfilaments show polarity; all of the subunits are oriented in the same direction.
This polarity influences the direction of microfilament elongation; assembly usually proceeds more readily at one end of the growing microfilament, whereas disassembly is favored at the other end.
Cytoskeletal Filaments Adapt to Form Dynamic or Stable Structures
Cytoskeletal systems are dynamic and adaptable, organized more like ant trails than interstate highways. A single trail of ants may persist for many hours, extending from the ant nest to a delectable picnic site, but the individual ants within the trail are anything but static. If the ant scouts find a new and better source of food, or if the picnickers clean up and leave, the dynamic structure adapts with astonishing rapidity. In a similar way, large-scale cytoskeletal structures can change or persist, according to need, lasting for lengths of time ranging from less than a minute up to the cell’s lifetime. But the individual macromolecular components that make up these structures are in a constant state of flux. Thus, like the alteration of an ant trail, a structural rearrangement in a cell requires little extra energy when conditions change. Regulation of the dynamic behavior and assembly of cytoskeletal filaments allows eukaryotic cells to build an enormous range of structures from the three basic filament systems.


The micrographs above illustrate some of these structures. Actin filaments underlie the plasma membrane of animal cells, providing strength and shape to its thin lipid bilayer. They also form many types of cell-surface projections. Some of these are dynamic structures, such as the lamellipodia and filopodia that cells use to explore territory and move around. More stable arrays allow cells to brace themselves against an underlying substratum and enable muscle to contract. The regular bundles of stereocilia on the surface
of hair cells in the inner ear contain stable bundles of actin filaments that tilt as rigid rods in response to sound, and similarly organized microvilli on the surface of intestinal epithelial cells vastly increase the apical cell-surface area to enhance nutrient absorption. In plants, actin filaments drive the rapid streaming of cytoplasm inside cells.Microtubules, which are frequently found in a cytoplasmic array that extends to the cell periphery, can quickly rearrange themselves to form a bipolar mitotic spindle during cell division. They can also form cilia, which function as motile whips or sensory devices on the surface of the cell, or tightly aligned bundles that serve as tracks for the transport of materials down long neuronal axons. In plant cells, organized arrays of microtubules help to direct the pattern of cell wall synthesis, and in many protozoans they form the framework upon which the entire cell is built. Intermediate filaments line the inner face of the nuclear envelope, forming a protective cage for the cell’s DNA; in the cytosol, they are twisted into strong cables that can hold epithelial cell sheets together or help nerve cells to extend long and robust axons, and they allow us to form tough appendages such as hair and fingernails. An important and dramatic example of rapid reorganization of the cytoskeleton occurs during cell division, as shown below for a fibroblast growing in a tissue-culture dish.:

After the chromosomes have replicated, the interphase microtubule array that spreads throughout the cytoplasm is reconfigured into the bipolar mitotic spindle, which segregates the two copies of each chromosome into daughter nuclei. At the same time, the specialized actin structures that enable the fibroblast to crawl across the surface of the dish rearrange so that the cell stops moving and assumes a more spherical shape. Actin and its associated motor protein myosin then form a belt around the middle of the cell, the contractile ring, which constricts like a tiny muscle to pinch the cell in two. When division is complete, the cytoskeletons of the two daughter fibroblasts reassemble into theirinterphase structures to convert the two rounded-up daughter cells into smaller versions of the flattened, crawling mother cell. Many cells require rapid cytoskeletal rearrangements for their normal functioning during interphase as well.
The Cytoskeleton Determines Cellular Organization and Polarity
In cells that have achieved a stable, differentiated morphology—such as mature neurons or epithelial cells—the dynamic elements of the cytoskeleton must also provide stable, large-scale structures for cellular organization. On specialized epithelial cells that line organs such as the intestine and the lung, cytoskeletal-based cell-surface protrusions including microvilli and cilia are able to maintain a constant location, length, and diameter over the entire lifetime of the cell. For the actin bundles at the cores of microvilli on intestinal epithelial cells, this is only a few days. But the actin bundles at the cores of stereocilia on the hair cells of the inner ear must maintain their stable organization for the entire lifetime of the animal, since these cells do not turn over. Nonetheless, the individual actin filaments remain strikingly dynamic and are continuously remodeled and replaced every 48 hours, even within stable cell-surface structures that persist for decades. Besides forming stable, specialized cell-surface protrusions, the cytoskeleton is also responsible for large-scale cellular polarity, enabling cells to tell the difference between top and bottom, or front and back. The large-scale polarity information conveyed by cytoskeletal organization is often maintained over the lifetime of the cell. Polarized epithelial cells use organized arrays of microtubules, actin filaments, and intermediate filaments to maintain the critical differences between the apical surface and the basolateral surface. They also must maintain strong adhesive contacts with one another to enable this single layer of cells to serve as an effective physical barrier

Filaments Assemble from Protein Subunits That Impart Specific Physical and Dynamic Properties
Cytoskeletal filaments can reach from one end of the cell to the other, spanning tens or even hundreds of micrometers. Yet the individual protein molecules that form the filaments are only a few nanometers in size. The cell builds the filaments by assembling large numbers of the small subunits, like building a skyscraper out of bricks. Because these subunits are small, they can diffuse rapidly in the cytosol, whereas the assembled filaments cannot. In this way, cells can undergo rapid structural reorganizations, disassembling filaments at one site and reassembling them at another site far away. Actin filaments and microtubules are built from subunits that are compact and globular—actin subunits for actin filaments and tubulin subunits for microtubules— whereas intermediate filaments are made up of smaller subunits that are themselves elongated and fibrous. All three major types of cytoskeletal filaments form as helical assemblies of subunits that self-associate, using a combination of end-to-end and side-to-side protein contacts. Differences in the structures of the subunits and the strengths of the attractive forces between them produce important differences in the stability and mechanical properties of each type of filament. Whereas covalent linkages between their subunits hold together the backbones of many biological polymers—including DNA, RNA, and proteins—it is weak noncovalent interactions that hold together the three types of cytoskeletal polymers. Consequently, their assembly and disassembly can occur rapidly, without covalent bonds being formed or broken.
The subunits of actin filaments and microtubules are asymmetrical and bind to one another head-to-tail so that they all point in one direction. This subunit polarity gives the filaments structural polarity along their length, and makes the two ends of each polymer behave differently. In addition, actin and tubulin subunits are both enzymes that catalyze the hydrolysis of a nucleoside triphosphate— ATP and GTP, respectively. The energy derived from nucleotide hydrolysis enables the filaments to remodel rapidly. By controlling when and where actin and microtubules assemble, the cell harnesses the polar and dynamic properties of these filaments to generate force in a specific direction, to move theleading edge of a migrating cell forward, for example, or to pull chromosomes apart during cell division. In contrast, the subunits of intermediate filaments are symmetrical, and thus do not form polarized filaments with two different ends. Intermediate filament subunits also do not catalyze the hydrolysis of nucleotides. Nevertheless, intermediate filaments can be disassembled rapidly when required. In mitosis , for example, kinases phosphorylate the subunits, leading to their dissociation. Cytoskeletal filaments in living cells are not built by simply stringing subunits together in single file. A thousand tubulin subunits lined up end-to-end, for example, would span the diameter of a small eukaryotic cell, but a filament formed in this way would lack the strength to avoid breakage by ambient thermal energy, unless each subunit in the filament was bound extremely tightly to its two neighbors. Such tight binding would limit the rate at which the filaments could disassemble, making the cytoskeleton a static and less useful structure. To provide both strength and adaptability, microtubules are built of 13 protofilaments—linear strings of subunits joined end-to-end—that associate with one another laterally to form a hollow cylinder. The addition or loss of a subunit at the end of one protofilament makes or breaks a small number of bonds. In contrast, loss of a subunit from the middle of the filament requires breaking many more bonds, while breaking it in two requires breaking bonds in multiple protofilaments all at the same time

The greater energy required to break multiple noncovalent bonds simultaneously allows microtubules to resist thermal breakage, while allowing rapid subunit addition and loss at the filament ends. Helical actin filaments are much thinner and therefore require much less energy to break. However, multiple actin filaments are often bundled together inside cells, providing mechanical strength, while allowing dynamic behavior of filament ends. As with other specific protein–protein interactions, many hydrophobic interactions and noncovalent bonds hold the subunits in a cytoskeletal filament togethe . The locations and types of subunit–subunit contacts differ for the different filaments. Intermediate filaments, for example, assemble by forming strong lateral contacts between α-helical coiled-coils, which extend over most of the length of each elongated fibrous subunit. Because the individual subunits are staggered in the filament, intermediate filaments form strong, ropelike structures that tolerate stretching and bending to a greater extent than do either actin filaments or microtubules
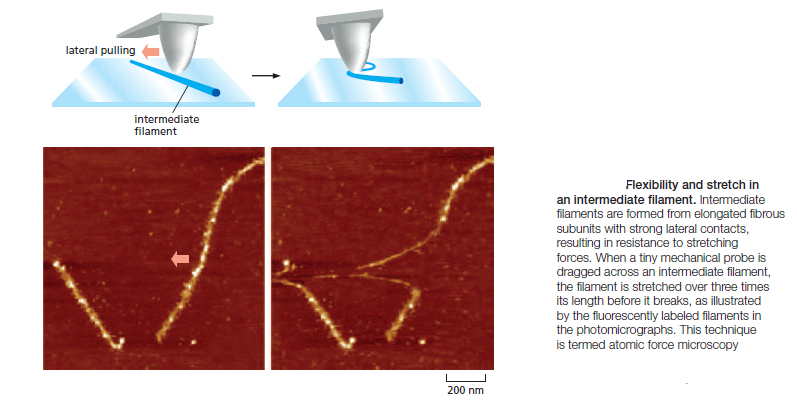
Accessory Proteins and Motors Regulate Cytoskeletal Filaments
The cell regulates the length and stability of its cytoskeletal filaments, as well as their number and geometry. It does so largely by regulating their attachments to one another and to other components of the cell, so that the filaments can form a wide variety of higher-order structures. Direct covalent modification of the filament subunits regulates some filament properties, but most of the regulation is performed by hundreds of accessory proteins that determine the spatial distribution and the dynamic behavior of the filaments, converting information received through signaling pathways into cytoskeletal action. These accessory proteins bind to the filaments or their subunits to determine the sites of assembly of new filaments, to regulate the partitioning of polymer proteins between filament and subunit forms, to change the kinetics of filament assembly and disassembly, to harness energy to generate force, and to link filaments to one another or to other cell structures such as organelles and the plasma membrane. In these processes, the accessory proteins bring cytoskeletal structure under the control of extracellular and intracellular signals, including those that trigger the dramatic transformations of the cytoskeleton that occur during each cell cycle. Acting together, the accessory proteins enable a eukaryotic cell to maintain a highly organized but flexible internal structure and, in many cases, to move. Among the most fascinating proteins that associate with the cytoskeleton are the motor proteins. These proteins bind to a polarized cytoskeletal filament and
use the energy derived from repeated cycles of ATP hydrolysis to move along it. Dozens of different motor proteins coexist in every eukaryotic cell. They differ in the type of filament they bind to (either actin or microtubules), the direction in which they move along the filament, and the “cargo” they carry. Many motor proteins carry membrane-enclosed organelles—such as mitochondria, Golgi stacks, or secretory vesicles—to their appropriate locations in the cell. Other motor proteins cause cytoskeletal filaments to exert tension or to slide against each other, generating the force that drives such phenomena as muscle contraction, ciliary beating, and cell division. Cytoskeletal motor proteins that move unidirectionally along an oriented polymer track are reminiscent of some other proteins and protein complexes , such as DNA and RNA polymerases, helicases, and
ribosomes. All of these proteins have the ability to use chemical energy to propel themselves along a linear track, with the direction of sliding dependent on the structural polarity of the track. All of them generate motion by coupling nucleoside triphosphate hydrolysis to a large-scale conformational change
Bacterial Cell Organization and Division Depend on Homologs of Eukaryotic Cytoskeletal Proteins
While eukaryotic cells are typically large and morphologically complex, bacterial cells are usually only a few micrometers long and assume simple shapes such as spheres or rods. Bacteria also lack elaborate networks of intracellular membrane- enclosed organelles. Historically, biologists assumed that a cytoskeleton was not necessary in such simple cells. We now know, however, that bacteria contain homologs of all three of the eukaryotic cytoskeletal filaments. Furthermore, bacterial actins and tubulins are more diverse than their eukaryotic versions, both in the types of assemblies they form and in the functions they carry out. Nearly all bacteria and many archaea contain a homolog of tubulin called FtsZ,which can polymerize into filaments and assemble into a ring (called the Z-ring) at the site where the septum forms during cell division

The bacterial FtsZ protein, a tubulin homolog in prokaryotes.
(A) A band of FtsZ protein forms a ring in a dividing bacterial cell. This ring has been labeled by fusing the FtsZ protein to green fluorescent protein (GFP), which allows it to be observed in living E. coli cells with a fluorescence microscope.
(B) FtsZ filaments and circles, formed in vitro, as visualized using electron microscopy.
(C) Dividing chloroplasts (red) from a red alga also cleave using a protein ring made from FtsZ (yellow).
Although the Z-ring persists for many minutes, the individual filaments within it are highly dynamic, with an average filament half-life of about thirty seconds. As the bacterium
divides, the Z-ring becomes smaller until it has completely disassembled. FtsZ filaments in the Z-ring are thought to generate a bending force that drives the membrane invagination necessary to complete cell division. The Z-ring may also serve as a site for localization of enzymes required for building the septum between the two daughter cells.Many bacteria also contain homologs of actin. Two of these, MreB and Mbl, are found primarily in rod-shaped or spiral-shaped cells where they assemble to form dynamic patches that move circumferentially along the length of the cell
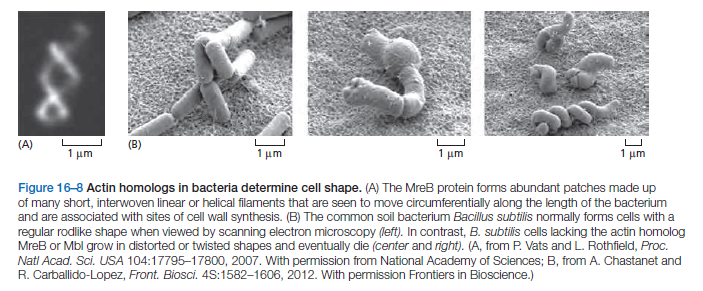
These proteins contribute to cell shape by serving as a scaffold to direct the synthesis of the peptidoglycan cell wall, in much the same way that microtubules help organize the synthesis of the cellulose cell wall in higher plant cells . As with FtsZ, MreB and Mbl filaments are highly dynamic, with half-lives of a few minutes, and nucleotide hydrolysis accompanies the polymerization process. Mutations disrupting MreB or Mbl expression cause extreme abnormalities in cell shape and defects in chromosome segregation (Figure
B above).
ACTIN AND ACTIN-BINDING PROTEINS
The actin cytoskeleton performs a wide range of functions in diverse cell types. Each actin subunit, sometimes called globular or G-actin, is a 375-amino-acid polypeptide carrying a tightly associated molecule of ATP or ADP

The structures of an actin monomer and actin filament.
(A) The actin monomer has a nucleotide (either ATP or ADP) bound in a deep cleft in the center of the molecule.
(B) Arrangement of monomers in a filament consisting of two protofilaments, held together by lateral contacts, which wind around each other as two parallel strands of a helix, with a twist repeating every 37 nm. All the subunits within the filament have the same orientation.
(C) Electron micrograph of negatively stained actin filament.
Actin is extraordinarily well conserved among eukaryotes. The amino acid sequences of actins from different eukaryotic species are usually about 90% identical. Small variations in actin amino acid sequence can cause significant functional differences: In vertebrates, for example, there are three isoforms of actin, termed α, β, and γ, that differ slightly in their amino acid sequences and have distinct functions. α-Actin is expressed only in muscle cells, while β- and γ-actins are found together in almost all non-muscle cells.
Actin Subunits Assemble Head-to-Tail to Create Flexible, Polar Filaments
Actin subunits assemble head-to-tail to form a tight, right-handed helix, forming a structure about 8 nm wide called filamentous or F-actin (Figure B and C above). Because the asymmetrical actin subunits of a filament all point in the same direction, filaments are polar and have structurally different ends: a slower-growing minus end and a faster-growing plus end. The minus end is also referred to as the “pointed end” and the plus end as the “barbed end,” because of the “arrowhead” appearance of the complex formed between actin filaments and the motor protein myosin

Within the filament, the subunits are positioned with their nucleotide-binding cleft directed toward the minus end. Individual actin filaments are quite flexible. The stiffness of a filament can be characterized by its persistence length, the minimum filament length at which random thermal fluctuations are likely to cause it to bend. The persistence length of an actin filament is only a few tens of micrometers. In a living cell, however, accessory proteins cross-link and bundle the filaments together, making largescale actin structures that are much more rigid than an individual actin filament
Nucleation Is the Rate-Limiting Step in the Formation of Actin Filaments
The regulation of actin filament formation is an important mechanism by which cells control their shape and movement. Small oligomers of actin subunits can assemble spontaneously, but they are unstable and disassemble readily because each monomer is bound to only one or two other monomers. For a new actin filament to form, subunits must assemble into an initial aggregate, or nucleus, that is stabilized by multiple subunit–subunit contacts and can then elongate rapidly by addition of more subunits. This process is called filament nucleation. Many features of actin nucleation and polymerization have been studied with purified actin in a test tube
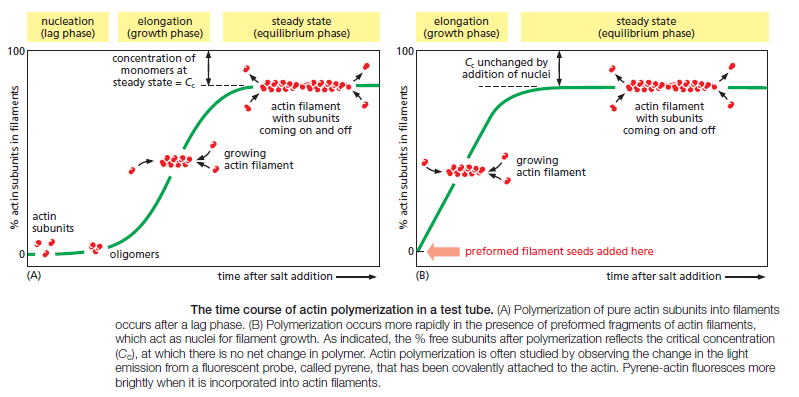
The instability of smaller actin aggregates creates a kinetic barrier to nucleation. When polymerization is initiated, this barrier results in a lag phase during which no filaments are observed. During this lag phase, however, a few of the small, unstable aggregates succeed in making the transition to a more stable form that resembles an actin filament. This leads to a phase of rapid filament elongation during which subunits are added quickly to the ends of the nucleated filaments (Figure A above). Finally, as the concentration
of actin monomers declines, the system approaches a steady state at which the rate of addition of new subunits to the filament ends exactly balances the rate of subunit dissociation. The concentration of free subunits left in solution at this point is called the critical concentration, Cc. The value of the critical concentration is equal to the rate constant for subunit loss divided by the rate constant for subunit addition—that is, Cc = koff/kon, which is equal to the dissociation constant, Kd, and the inverse of the equilibrium constant, K. In a test tube, the Cc for actin polymerization—that is, the free actin monomer concentration at which the fraction of actin in the polymer stops increasing—is about 0.2 μM. Inside the cell, the concentration of unpolymerized actin is much higher than this, and the cell has mechanisms to prevent most of its monomeric actin from assembling into filamentsr.
Question : how did these mechanisms emerge ? how did the function to assemble the right amount of actin filaments emerge ? How did the monomeric units emerge with the right fit and the right size and the right quantity ? trial and error ? had these filaments not have to be present right from the start, in order to get the right cell shape ?
The lag phase in filament growth is eliminated if preexisting seeds (such as fragments of actin filaments that have been chemically cross-linked) are added to the solution at the beginning of the polymerization reaction (Figure B above). The cell takes great advantage of this nucleation requirement: it uses special proteins to catalyze filament nucleation at specific sites, thereby determining the location at which new actin filaments are assembled.
Actin Filaments Have Two Distinct Ends That Grow at Different Rates
Due to the uniform orientation of asymmetric actin subunits in the filament, the structures at its two ends are different. This orientation makes the two ends of each polymer different in ways that have a profound effect on filament growth rates. The kinetic rate constants for actin subunit association and dissociation— kon and koff, respectively—are much greater at the plus end than the minus end. This can be seen when an excess of purified actin monomers is allowed to assemble onto polarity-marked filaments—the plusend of the filament elongates up to ten times faster . If filaments are rapidly diluted so that the free subunit concentration drops below the critical concentration, the plus end also
depolymerizes faster. It is important to note, however, that the two ends of an actin filament have the same net affinity for actin subunits, if all of the subunits are in the same nucleotide state. Addition of a subunit to either end of a filament of n subunits results in a filament of n + 1 subunits. Thus, the free-energy difference, and therefore the equilibrium constant (and the critical concentration), must be the same for addition of subunits at either end of the polymer. In this case, the ratio of the rate constants,
koff/kon, must be identical at the two ends, even though the absolute values of these rate constants are very different at each end. The cell takes advantage of actin filament dynamics and polarity to do mechanical work. Filament elongation proceeds spontaneously when the free-energy change (ΔG) for addition of the soluble subunit is less than zero. This is the case when the concentration of subunits in solution exceeds the critical concentration. A cell can couple an energetically unfavorable process to this spontaneous
process; thus, the cell can use free energy released during spontaneous filament polymerization to move an attached load. For example, by orienting the fast-growing plus ends of actin filaments toward its leading edge, a motile cell can push its plasma membrane forward.
ATP Hydrolysis Within Actin Filaments Leads to Treadmilling at Steady State
Thus far in our discussion of actin filament dynamics, we have ignored the critical fact that actin can catalyze the hydrolysis of the nucleoside triphosphate ATP. For free actin subunits, this hydrolysis proceeds very slowly; however, it is accelerated when the subunits are incorporated into filaments. Shortly after ATP hydrolysis occurs, the free phosphate group is released from each subunit, but the ADP remains trapped in the filament structure. Thus, two different types of filament structures can exist, one with the “T form” of the nucleotide bound (ATP), and one with the “D form” bound (ADP). When the nucleotide is hydrolyzed, much of the free energy released by cleavage of the phosphate–phosphate bond is stored in the polymer. This makes the free-energy change for dissociation of a subunit from the D-form polymer more negative than the free-energy change for dissociation of a subunit from the T-form polymer. Consequently, the ratio of koff/kon for the D-form polymer, which is numerically equal to its critical concentration [Cc(D)], is larger than the corresponding ratio for the T-form polymer. Thus, Cc(D) is greater than Cc(T). At certain concentrations of free subunits, D-form polymers will therefore shrink while T-form polymers grow.
In living cells, most soluble actin subunits are in the T form, as the free concentration of ATP is about tenfold higher than that of ADP. However, the longer the time that subunits have been in the actin filament, the more likely they are to have hydrolyzed their ATP. Whether the subunit at each end of a filament is in the T or the D form depends on the rate of this hydrolysis compared with the rate of subunit addition. If the concentration of actin monomers is greater than the critical concentration for both the T-form and D-form polymer, then subunits will add to the polymer at both ends before the nucleotides in the previously added subunits are hydrolyzed; as a result, the tips of the actin filament will remain in the T form. On the other hand, if the subunit concentration is less than the critical concentrations for both the T-form and D-form polymer, then hydrolysis may occur before the next subunit is added and both ends of the filament will be in the D form and will shrink. At intermediate concentrations of actin subunits, it is possible for the rate of subunit addition to be faster than nucleotide hydrolysis at the plus end, but slower than nucleotide hydrolysis at the minus end. In this case, the plus end of the filament remains in the T conformation, while the minus end adopts the D conformation. The filament then undergoes a net addition of subunits at the plus end, while simultaneously losing subunits from the minus end. This leads to the remarkable property of filament treadmilling

At a particular intermediate subunit concentration, the filament growth at the plus end exactly balances the filament shrinkage at the minus end. Under these conditions, the subunits cycle rapidly between the free and filamentous states

while the total length of the filament remains unchanged. This “steady-state treadmilling requires a constant consumption of energy in the form of ATP hydrolysis. Within a cell, actin behavior is regulated by numerous accessory proteins that bind actin monomers or filaments
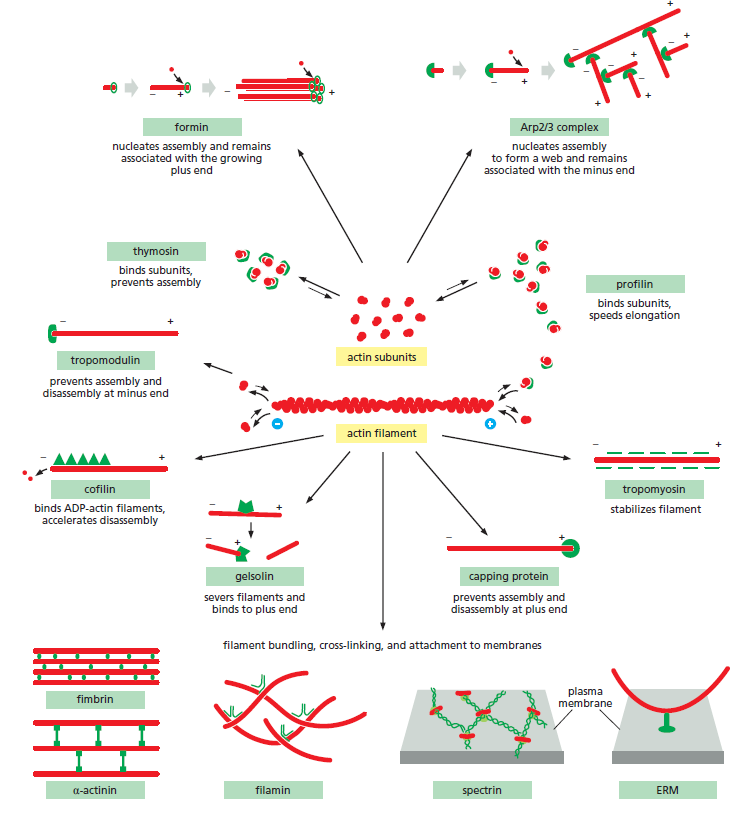
Some of the major accessory proteins of the actin cytoskeleton. Except for the myosin motor proteins, an example of each major type is shown. Each of these is discussed in the text. However, most cells contain more than a hundred different actin-binding proteins, and it is likely that there are important types of actin-associated proteins that are not yet recognized