1
What is embodied life?
In my previous book, On the Origin of Life and Virus World by means of an Intelligent Designer, I described cells as chemical factories, not just analogously to human-made factories, but in a literal sense. In order for considering alive, cells have to be able to reproduce and self-replicate, perform metabolic reactions, where chemicals are transformed, and processed by going through complex enzyme-induced transformations. Able to take up materials, recycle, and expel waste products, grow and develop, evolve, that is to adapt to the environment, and speciate. They are complex, requiring millions of components, like monomers, polymers, proteins, cell membranes, etc. Cells must be able to keep a homeostatic milieu and maintain inner balance, and stable internal conditions. They need energy in order to be able to perform their cellular functions. And be able to respond to external stimuli. Living cells depend on complex information data storage, transmission, transcription, translation, decoding, and expression of that information used to direct the assembly and operation of the cell factory. It must be able to replicate and transmit the genetic epigenetic material to the next generation, to the daughter cells. Cells would not be able to survive without the ability of error detection and repair.
Y.Sebag: Just briefly, to get a feel for what cells need to do, let us consider the basic autonomous cell whose task is to reproduce and synthesize the parts it needs from raw materials.
1. Information System - Building something which can reproduce and synthesize its own parts from raw materials requires a coordinated series of steps. Chemicals cannot do this. On their own, they just combine chaotically or crystallize into regular patterns such as in snowflakes. Hence, there must be information (ex. RNA or the like) storing the information to orchestrate the assembly.
2. Energy System - information by itself is useless. Implementing the instructions requires energy. A system that cannot generate or source energy just drifts chaotically or crystallizes into simple forms, forced to follow the path of least resistance. Hence, a system of producing or sourcing energy is necessary along with subsystems of distribution and management of that energy so that it goes to the proper place.
3. Copy System - in order to reproduce itself, the device must be able to implement the instructions of the information system using the energy system. This includes the ability to rebuild all critical infrastructure such as the information and energy systems and even the copy system itself.
4. Growth System - Without a growth system, the device will reduce itself every time it reproduces and vanish to zero-size after a few generations. This growth system necessitates subsystems of ingestion of materials from the outside world, processing of those materials, and assembling those materials into the necessary parts. This alone is a formidable chemical factory.
5. Transportation System - the materials must be moved to the proper places. Hence, a transportation system is needed for transporting raw materials and products from one place to another within the cell. Likewise, a system for managing the incoming of raw materials and outgoing of waste materials of all these chemical reactions.
6. Timing System - the growth system must also be coordinated with the reproduction system. Otherwise, if reproduction occurs faster than growth, it will reduce size faster than it grows and vanishes after a few generations. Hence, a timing or feedback mechanism is needed.
7. Communication System - signalling is needed to coordinate all the tasks so that they all work together. The reproduction system won't work without coordination with the growth and power systems. Likewise, the power system by itself is useless without the growth and reproduction systems. Only when all the systems and "circuitry" are in place and the power is turned on is there hope for the various interdependent tasks to start working together. Otherwise, it is like turning on a computer which has no interconnections between the power supply, CPU, memory, hard drive, video, operating system, etc - nothing to write home about.1
Cells are full of chemical factories and machines in a literal sense
Unguided events, without the influence of an intelligent agent, have not been demonstrated to assemble complex chemical factories driven by software programs, which in the context of biology, are the instructions encoded in DNA and executed by cellular machinery. Cells are often described as information-driven chemical factories due to their ability to process, store, and utilize genetic information to carry out complex biological processes. Biological systems, such as cells, are incredibly complex. They consist of intricate molecular networks and regulatory mechanisms that enable them to perform a wide range of functions necessary for life. The organization and functionality of these systems require precise coordination and control, often involving multiple interacting components. From an informational perspective, cells contain and process vast amounts of genetic information that govern the assembly and operation of their molecular machinery. Within cells, genetic information is stored in the form of DNA (or RNA in some cases) and is transcribed and translated into functional molecules, such as proteins. This genetic code is essentially a set of instructions that guide the synthesis of specific proteins, which are key players in the cell's structure, metabolism, and signaling processes. The storage, transmission, and interpretation of genetic information require complex molecular machinery and cellular processes. When referring to cells as "information-driven chemical factories," an analogy is often drawn between the information processing in cells and the operation of software programs in computers. In both cases, information is stored, processed, and used to execute specific functions. Cells utilize sophisticated molecular machinery and biochemical processes to interpret and execute the instructions encoded in the genetic code, similar to how computers use hardware and software to process and execute instructions. The emergence of complex biological systems, driven by information and executed through intricate molecular processes, is best explained by the involvement of an intelligent agent. The probability of such complex systems arising solely through random, unguided processes is highly unlikely.
Premise 1: Unguided events, without the influence of an intelligent agent, have not been demonstrated to assemble complex chemical factories driven by software programs, which in the context of biology, are the instructions encoded in DNA and executed by cellular machinery. Cells are information-driven chemical factories due to their ability to process, store, and utilize genetic information to carry out complex biological processes. They consist of intricate molecular networks and regulatory mechanisms that enable them to perform a wide range of functions necessary for life.
Premise 2: Genetic information is stored in the form of DNA (or RNA) within cells and is transcribed and translated into functional molecules, such as proteins. This genetic code acts as a set of instructions guiding the synthesis of specific proteins, which play key roles in the cell's structure, metabolism, and signaling processes. The storage, transmission, and interpretation of genetic information within cells require complex molecular machinery and cellular processes. Cells utilize sophisticated molecular machinery and biochemical processes to interpret and execute the instructions encoded in the genetic code, similar to how computers use hardware and software to process and execute instructions.
Conclusion: The emergence of complex biological systems, driven by information and executed through intricate molecular processes, is best explained by the involvement of an intelligent agent. The precise coordination, functionality, and storage of vast amounts of genetic information within cells, along with their ability to carry out a wide range of biological functions, are highly complex phenomena that have not been demonstrated to arise solely through random, unguided processes.
Cells can be thought of as literal chemical factories and machines because they are constantly performing biochemical reactions and processes to maintain their functions and sustain life. They take in raw materials from their environment and convert them into various products that the cell needs to survive, such as proteins, lipids, and energy.
Each cell can be seen as a complex network of interlocking assembly lines, with large protein machines and complexes working together in a highly coordinated manner. For example, the nucleolus is a large factory where non-coding RNAs are transcribed, processed, and assembled with proteins to form ribonucleoprotein complexes. The endoplasmic reticulum serves as a factory for the production of almost all of the cell's lipids, and in response to DNA damage, repair factories are formed where damaged DNA is brought together and repaired.
Protein assemblies in cells contain highly coordinated moving parts, with intermolecular collisions restricted to a small set of possibilities, similar to machines invented by humans. These assemblies contain ordered conformational changes in one or more proteins driven by nucleoside triphosphate hydrolysis or other sources of energy, allowing them to function in a polarized fashion along a filament or nucleic acid strand, increase the fidelity of biological reactions, or catalyze the formation of protein complexes.
The complexity of cells can be difficult to grasp, but imagining the size of a cell magnified ten thousand million times gives a sense of the scale of the processes and structures at work. At that size, a cell would have a radius of 200 miles, which is about ten times the size of New York City. Even with that much space, the required number of buildings to host the factories and machines that cells need would greatly exceed the number of buildings in the city.
B.Alberts (2022): The surface of our planet is populated by living things—organisms—curious, intricately organized chemical factories that take in matter from their surroundings and use these raw materials to generate copies of themselves. Although all cells function as biochemical factories of a broadly similar type, many of the details of their small-molecule transactions differ. All cells operate as biochemical factories, driven by the free energy released in a complicated network of chemical reactions. Each cell can be viewed as a tiny chemical factory, performing many millions of reactions every second. We can view RNA polymerase II in its elongation mode as an RNA factory that not only moves along the DNA synthesizing an RNA molecule but also processes the RNA that it produces. The nucleolus can be thought of as a large factory at which different noncoding RNAs are transcribed, processed, and assembled with proteins to form a large variety of ribonucleoprotein complexes. mRNA production is made more efficient in the nucleus by an aggregation of the many components needed for transcription and pre-mRNA processing, thereby producing a specialized biochemical factory. The extensive ER network serves as a factory for the production of almost all of the cell’s lipids. In response to DNA damage, they rapidly converge on the sites of DNA damage, become activated, and form “repair factories” where many lesions are apparently brought together and repaired. The formation of these factories probably results from many weak interactions between different repair proteins and between repair proteins and damaged DNA. 2
B.Alberts (1998): We can walk and we can talk because the chemistry that makes life possible is much more elaborate and sophisticated than anything we students had ever considered. Proteins make up most of the dry mass of a cell. But instead of a cell dominated by randomly colliding individual protein molecules, we now know that nearly every major process in a cell is carried out by assemblies of 10 or more protein molecules. And, as it carries out its biological functions, each of these protein assemblies interacts with several other large complexes of proteins. Indeed, the entire cell can be viewed as a factory that contains an elaborate network of interlocking assembly lines, each of which is composed of a set of large protein machines. Consider, as an example, the cell cycle–dependent degradation of specific proteins that helps to drive a cell through mitosis. First, a large complex of about 10 proteins, the anaphase-promoting complex (APC), selects out a specific protein for polyubiquitination; this protein is then targeted to the proteasome's 19S cap complex formed from about 20 different subunits; and the cap complex then transfers the targeted protein into the barrel of the large 20S proteasome itself, where it is finally converted to small peptides. Why do we call the large protein assemblies that underlie cell function protein machines? Precisely because, like the machines invented by humans to deal efficiently with the macroscopic world, these protein assemblies contain highly coordinated moving parts. Within each protein assembly, intermolecular collisions are not only restricted to a small set of possibilities, but reaction C depends on reaction B, which in turn depends on reaction A—just as it would in a machine of our common experience. Underlying this highly organized activity are ordered conformational changes in one or more proteins driven by nucleoside triphosphate hydrolysis (or by other sources of energy, such as an ion gradient). Because the conformational changes driven in this way dissipate free energy, they generally proceed only in one direction. An earlier brief review emphasized how the directionality imparted by nucleoside triphosphate hydrolyses allows allosteric proteins to function in three different ways: as motor proteins that move in a polarized fashion along a filament or a nucleic acid strand; as proofreading devices or “clocks” that increase the fidelity of biological reactions by screening out poorly matched partners; and as assembly factors that catalyze the formation of protein complexes and are then recycled. 3
Magnifying a cell ten thousand million times, it would have a radius of 200 miles, about 10 times the size of New York City
Calling a cell a factory is an understatement. Magnifying the cell to a size of 200 miles, it would only contain the required number of buildings, hosting the factories to make the machines that it requires.
New York City has about 900.000 buildings, of which about 40.000 are in Manhattan, of which 7.000 are skyscrapers of high-rise buildings of at least 115 feet (35 m), of which at least 95 are taller than 650 feet (198 m).

Cells are an entire industrial park, where only the number of factories producing the machines used in the industrial park is of size at least 10 times the size of New York City, where each building is individually a factory comparable to the size of a skyscraper like the Twin Towers of the World Trade Center. Each tower hosts a factory that makes factories that make machines. A mammalian cell may harbor as many as 10 million ribosomes. The nucleolus is the factory that makes ribosomes, the factory that makes proteins, which are the molecular machines of the cell. The nucleolus can be thought of as a large factory at which different noncoding RNAs are transcribed, processed, and assembled with proteins to form a large variety of ribonucleoprotein complexes.
L. Lindahl (2022): Ribosome assembly requires synthesis and modification of its components, which occurs simultaneously with their assembly into ribosomal particles. The formation occurs by a stepwise ordered addition of ribosome components. The process is assisted by many assembly factors that facilitate and monitor the individual steps, for example by modifying ribosomal components, releasing assembly factors from an assembly intermediate, or forcing specific structural configurations. The quality of the ribosome population is controlled by a complement of nucleases that degrade assembly intermediates with an inappropriate structure and/or which constitute kinetic traps.4
Mitochondria, the powerhouse of the cell, can host up to 5000 ATP synthase energy turbines. Each human heart muscle cell contains up to 8,000 mitochondria. That means, in each of the human heart cells, there are up to 40 million ATP synthase energy turbines caring for the production of ATP, the energy currency in the cell.
M.Denton (2020): The miracle of the Cell : Pg.11
Where the cosmos feels infinitely large and the atomic realm infinitely small, the cell feels infinitely complex. They appear in so many ways supremely fit to fulfill their role as the basic unit of biological life.
Pg. 329.
We would see [in cells] that nearly every feature of our own advanced machines had its analog in the cell: artificial languages and their decoding systems, memory banks for information storage and retrieval, elegant control systems regulating the automated assembly of parts and components, error fail-safe and proof-reading devices utilized for quality control, assembly processes involving the principle of prefabrication and modular construction. In fact, so deep would be the feeling of deja-vu, so persuasive the analogy, that much of the terminology we would use to describe this fascinating molecular reality would be borrowed from the world of late-twentieth-century technology.
“What we would be witnessing would be an object resembling an immense automated factory, a factory larger than a city and carrying out almost as many unique functions as all the manufacturing activities of man on earth. However, it would be a factory that would have one capacity not equaled in any of our own most advanced machines, for it would be capable of replicating its entire structure within a matter of a few hours. To witness such an act at a magnification of one thousand million times would be an awe-inspiring spectacle.”5
M. Denton (1985) Evolution, a theory in crisis:
To grasp the reality of life as it has been revealed by molecular biology, we must magnify a cell a thousand million times until it is twenty kilometres in diameter and resembles a giant airship large enough to cover a great city like London or New York. What we would then see would be an object of unparalleled complexity and adaptive design. On the surface of the cell we would see millions of openings, like the port holes of a vast space ship, opening and closing to allow a continual stream of materials to flow in and out. If we were to enter one of these openings we would find ourselves in a world of supreme technology and bewildering complexity. We would see endless highly organized corridors and conduits branching in every direction away from the perimeter of the cell, some leading to the central memory bank in the nucleus and others to assembly plants and processing units. The nucleus itself would be a vast spherical chamber more than a kilometre in diameter, resembling a geodesic dome inside of which we would see, all neatly stacked together in ordered arrays, the miles of coiled chains of the DNA molecules.
A huge range of products and raw materials would shuttle along all the manifold conduits in a highly ordered fashion to and from all the various assembly plants in the outer regions of the cell. We would wonder at the level of control implicit in the movement of so many objects down so many seemingly endless conduits, all in perfect unison. We would see all around us, in every direction we looked, all sorts of robot-like machines. We would notice that the simplest of the functional components of the cell, the protein molecules, were astonishingly, complex pieces of molecular machinery, each one consisting of about three thousand atoms arranged in highly organized 3-D spatial conformation... Yet the life of the cell depends on the integrated activities of thousands, certainly tens, and probably hundreds of thousands of different protein molecules.
We would see that nearly every feature of our own advanced machines had its analogue in the cell: artificial languages and their decoding systems, memory banks for information storage and retrieval, elegant control systems regulating the automated assembly of parts and components, error fail-safe and proof-reading devices utilized for quality control, assembly processes involving the principle of prefabrication and modular construction. In fact, so deep would be the feeling of deja-vu, so persuasive the analogy, that much of the terminology we would use to describe this fascinating molecular reality would be borrowed from the world of late twentieth-century technology.
What we would be witnessing would be an object resembling an immense automated factory, a factory larger than a city and carrying out almost as many unique functions as all the manufacturing activities of man on earth..6
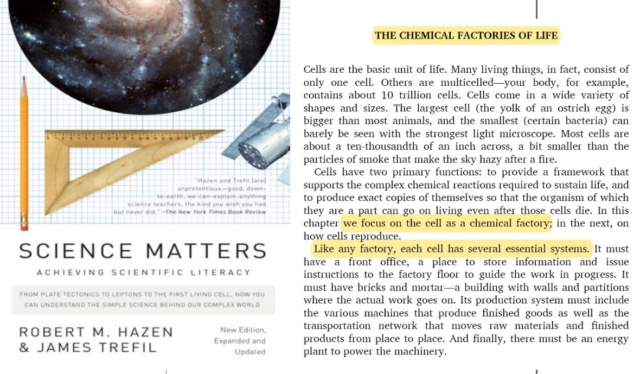
Robert M.Hazen Science matters (2009):
Pg.239 Cells act as chemical factories, taking in materials from the environment, processing them, and producing “finished goods” to be used for the cell’s own maintenance and for that of the larger organism of which they may be part. In a complex cell, materials are taken in through specialized receptors (“loading docks”), processed by chemical reactions governed by a central information system (“the front once”), carried around to various locations (“assembly lines”) as the work progresses, and finally sent back via those same receptors into the larger organism. The cell is a highly organized, busy place, whose many different parts must work together to keep the whole functioning. While proteins supervise the cell’s chemical factories, carbohydrates provide each factory’s fuel supply.
Pg. 242 Nucleic acids. These molecules (DNA and RNA) carry the blueprint that runs the cell’s chemical factories, and also are the vehicle for inheritance
Pg. 243 Carbohydrates. While proteins supervise the cell’s chemical factories, carbohydrates provide each factory’s fuel supply. The basic building blocks of carbohydrates are sugars—small ring-
Pg. 245 Like any factory, each cell has several essential systems. It must have a front office, a place to store information, and issue instructions to the factory door to guide the work in progress. It must have bricks and mortar—a building with walls and partitions where the actual work goes on. Its production system must include the various machines that produce finished goods as well as the transportation network that moves raw materials and finished products from place to place. And finally, there must be an energy plant to power the machinery.
Pg. 246 Cellular factories consist of walls, partitions, and loading docks.
Pg. 249 Every living thing is composed of one or more cells, each of which has a complex anatomy. A “generic” cell contains many structures and organelles—tiny chemical factories.
Pg. 263 The sequence of the bases along the double helix of DNA contains the genetic code—all the information a cell needs to reproduce itself and run its chemical factories, all the characteristics and quirks that make you unique.
Pg. 309 Shortly thereafter, the glucose is processed in cellular chemical factories to form part of the cellulose fibers that support each grass blade. The carbon atom has become an integral part of the structure of grass.7
Ben L. Feringa (2020): The miniaturization of complex physical and chemical systems is a key aspect of contemporary materials science. The bottom-up formation of dynamic structures with unusual properties has now been extended from the microscale to the nanoscale. Such extended dynamic structures are complemented by an increasing number of molecular species capable of transforming a physical or chemical stimulus into directional motion. These so-called artificial molecular machines (AMMs) are often regarded as molecular renderings of the macroscopic machines we experience in our daily lives — rotors, gears and cranks, for example. However, the inspiration for many AMMs is not from macroscopic man-made machines but, rather, from proteins or multi-protein complexes in biology that are capable of transforming energy into continuous, complex, structural motion. The process of vision, muscle contraction and bacterial flagellar movement are amazing examples of biological responsive systems. Biological molecular machines (BMMs) such as ATP synthase, ribosomes or myosin are structurally far more complex than any artificial molecular machines AMM made so far, and are an essential part of living systems. Embedded or immobilized within skeleton structures such as bilayer lipid membranes or larger protein complexes, BMMs are part of a cellular confinement in which their work is continuously synchronized with other machines of identical or different nature. Their functions are driven by chemical fuels such as ATP or electrochemical gradients and controlled by chemical or physical stimuli. Their main tasks involve intracellular, transmembrane and intercellular transport of reagents, as well as transformation of small, molecular building blocks into larger functional structures. A cell might thus be viewed as a complex molecular factory in which many different components are assembled, transformed, transported and disassembled. The dynamics of these processes at the molecular level are amplified by self-organization, cooperativity and synchronization, resulting in the living, moving organisms observed at the macroscopic scale. A modular building concept, periodical alignment and synchronization of individual dynamic components on a temporal and spatial domain are essential aspects of the performance of the whole system. Such organizational principles can also be found in macroscopic factories regardless of the difference in size, and they are considered fundamental principles in the design of cooperative dynamic systems of any size and composition. Nevertheless, biological systems strongly differ from man-made factories in certain aspects. BMMs and their complex assemblies are very versatile and selective in continuously producing a variety of complex molecules currently unobtainable by any man-made system. 8
Von Neumann's universal constructor: We cannot replicate the cell's self-reproduction technology
Imagine a hypothetical human-made truly autonomous self-replicating factory analogous to living cells. It would have to be capable of replicating itself and constructing a copy of itself, without external help. Able to detect raw materials in its surroundings, in the environment, that it needs, and prepare them to be transformed into the right form, so that import gates and mechanisms could import these materials into the factory inside. The daughter factory would require to get the entire information stored in the mother cell inherited. It would rely on conventional large-scale technology and automation.
M. Sipper (1998): We would need to be able to understand the fundamental information-processing principles and algorithms involved in self-replication, even independent of their physical realization.9
Replicators have been called "von Neumann machines" after John von Neumann, who first rigorously studied the idea. Von Neumann himself used the term universal constructor to describe such a self-replicating machine. For a factory or machine to make a duplicate copy it must employ a description of itself. This description, being a part of the original factory, must itself be prescribed by something else that is not itself. That is, it must come from the outside. Why? In order to describe something, one needs to be a conscious agent, able to do so. If the factory itself was not the conscious agent, being able to observe and describe itself, it must have been something else. I, as a human being, conscious, can observe and describe myself. A non-conscious "something" has never been seen as having these necessary cognitive and intelligent capabilities. That's why the origin of biological information is an unsolvable problem for naturalists. That's why the origin of the information to make the first living self-replicating cell cannot be solved unless there was a creator. Another salient point: Parts, subunits, or an agglomeration of building blocks do not comprehend how they could join to become part of a functional interlocked complex system. So in order to construct a self-replicating system composed of many interlocking parts, foresight is required, otherwise, the parts could either remain non-assembled, disintegrate, or, eventually, driven by random external forces, interact and assemble into a basically infinite number of nonfunctional chaotic aggregation states.
R. A. Freitas (2004): Von Neumann thus hit upon a deceptively simple architecture for machine replication. The machine would have four parts:
1. a constructor “A” that can build a machine “X” when fed explicit blueprints of that machine;
2. a blueprint copier “B”;
3. a controller “C” that controls the actions of the constructor and the copier, actuating them alternately; and finally
4. a set of blueprints φ(A + B + C) explicitly describing how to build a constructor, a controller, and a copier.
The entire replicator may therefore be described as (A + B + C) + φ(A + B + C.
Observers have noted that von Neumann’s early schema was later confirmed by subsequent research on the molecular biology of cellular reproduction, with von Neumann’s component “A” represented by the ribosomes and supporting cellular mechanisms, component “B” represented by DNA polymerase enzymes, component “C” represented by repressor and derepressor molecules and associated expression-control machinery in the cell, and finally component “φ(A + B + C)” represented by the genetic material DNA that carries the organism’s genome. (The correspondence is not complete: cells include additional complexities.) More importantly, the dual use of information — both interpreted and uninterpreted, as in von Neumann’s machine schema — was also found to be true for the information contained in DNA.10
M. Sipper (1998): A noteworthy distinction apparent in von Neumann’s model of self-replication is the double-faceted use of the information stored in the artificial genome: It first serves as instructions to be interpreted so as to construct a new universal constructor, after which this same genome is copied unmodified, to be attached to the new offspring constructor—so that it may replicate in its turn. This aspect is quite interesting in that it bears strong resemblance to the genetic mechanisms of transcription (copying) and translation (interpretation) employed by biological life—which was discovered during the decade following von Neumann’s work. Von Neumann’s model employs a complex transition rule, with the total number of cells composing the universal constructor estimated at between 50,000 and 200,000 (the literature seems to disagree on the exact number). In the years that followed its introduction a number of researchers had worked toward simplifying this system. In the late 1960s Codd reduced the number of states required for a self-replicating universal constructor-computer from 29 to 8. His self-replicating structure comprised about 100,000,000 cells. A few years later Devore simplified Codd’s system, devising a self-replicating automaton comprising about 100,000 cells.
Despite the complexity of von Neumann’s self-replicating universal constructor, a number of researchers have considered its implementation (or simulation) over the years. Signorini concentrated on the 29-state transition rule, discussing its implementation on a SIMD (single-instruction multiple-data) computer. Von Neumann’s constructor is divided into many functional blocks known as organs. In addition to implementing the transition rule, Signorini also presented the implementation of three such organs: a pulser, a decoder, and a periodic pulser. To date, Pesavento’s more recent work comes closest to a full simulation of von Neumann’s model. A computer simulation of the universal constructor—running on a standard workstation—even this comes short of realizing the full model: Self-replication is not demonstrated because the tape required to describe the constructor (i.e., the genome) is too large to simulate. 11
R. A. Freitas (2004): Penrose, quoting Kemeny, complained that the body of the von Neumann kinematic machine “would be a box containing a minimum of 32,000 constituent parts (likely to include rolls of tape, pencils, erasers, vacuum tubes, dials, photoelectric cells, motors, batteries, and other devices) and the ‘tail’ would comprise 150,000 [bits] of information.” Macroscale kinematic replicators will require a great deal of effort to design and to build, which may explain why so few working devices have been constructed to date,* despite popular interest. 12
Comment: A Von Neumann self-replicating machine has never been constructed because it is too complicated. Man, with all its intelligence, has failed. But, if abiogenesis is true, the emergence of self-replicating cells with a minimum of one million bits of information happened from randomly distributed, nonreplicating components by entirely non-intelligent unguided means.
A Self-Replicating Box
G. SEWELL (2021): To understand why human-engineered self-replicating machines are so far beyond current human technology, let’s imagine trying to design something as “simple” as a self-replicating cardboard box. Let’s place an empty cardboard box (A) on the floor, and to the right of it let’s construct a box (B) with a box-building factory inside it. I’m not sure exactly what the new box would need to build an empty box, but I assume it would at least have to have some metal parts to cut and fold the cardboard and a motor with a battery to power these parts. In reality, to be really self-replicating like living things, it would have to go get its own cardboard, so maybe it would need wheels and an axe to cut down trees and a small sawmill to make cardboard out of wood. But let’s be generous and assume humans are still around to supply the cardboard. Well, of course box B is not a self-replicating machine, because it only produces an empty box A.
So, to the right of this box, let’s build another box C which contains a fully automated factory that can produce box B’s. This is a much more complicated box, because this one must manufacture the metal parts for the machinery in box B and its motor and battery and assemble the parts into the factory inside B. In reality it needs to go mine some ore and smelt it to produce these metal parts, but again let’s be very generous and provide it all the metals and other raw materials it needs.
But box C would still not be a self-replicating machine, because it only produces the much simpler box B. So back to work, now we need to build a box D to its right with a fully automated factory capable of building box C’s with their box B factories. Well, you get the idea, and one begins to wonder if it is even theoretically possible to build a truly self-replicating machine. When we add technology to such a machine to bring it closer to the goal of reproduction, we only move the goalposts, because now we have a more complicated machine to reproduce. Yet we see such machines all around us in the living world.
If we keep adding boxes to the right, each with a fully automated factory that can produce the box to its left, it seems to me that the boxes would grow exponentially in complexity. But maybe I am wrong. Maybe they could be designed to converge eventually to a self-replicating box Z, although I can’t imagine how. 13
The self-assembly of a factory starting with unorganized raw materials has never been observed
When discussing the assembly of complex structures from raw materials "just laying around," we have to consider that solely the laws of physics and chemistry govern the behavior of these materials. The spontaneous self-assembly of complex factories or structures solely from raw materials without any external intervention is currently not well-documented or understood. In other words, it has never been demonstrated to be possible. The spontaneous assembly of a complex factory or structure through unguided means, without any external intelligence or intervention, has not been demonstrated or observed in scientific experiments or natural processes. While self-assembly and self-organization are observed in various systems, they often involve specific conditions, preexisting structures, or programmed interactions between components. When we talk about self-assembly and self-organization, it's important to understand that these processes typically occur within certain contexts and conditions. For example:
In living organisms, self-assembly processes are observed in various structures, such as the formation of cellular membranes, the assembly of protein complexes, or the organization of DNA into chromosomes. However, these processes rely on preexisting biological components, such as proteins, lipids, or nucleic acids, which have specific molecular interactions and are governed by biological mechanisms. The assembly and organization of these structures are guided by genetic information and cellular processes, involving complex networks of chemical reactions and molecular interactions.
In the field of nanotechnology, scientists have developed self-assembling systems at the molecular scale. These systems often involve specially designed molecules or nanoparticles that possess specific properties or functional groups. Through these properties, the components can interact and align in a way that facilitates self-assembly. The process may require specific environmental conditions, such as a particular solvent or temperature range, to trigger the self-assembly. Therefore, while self-organization is observed, it still relies on the design and manipulation of the components and their surrounding environment.
In synthetic systems, researchers have explored self-assembly processes using engineered components. For example, in robotics, researchers have developed small robotic units that can autonomously assemble into larger structures or perform collective tasks. However, these systems typically involve programmed interactions and behaviors. The individual units may have sensors, communication capabilities, or predefined rules that govern their assembly and coordination. They are designed with specific capabilities and functionalities to enable self-assembly under controlled conditions.
Observe the keywords here: guided by genetic information, and the involvement of programmed interactions. Generating information and programmed interactions typically require the involvement of a programmer or an intelligent agent. In the context of self-assembly and self-organization, the patterns, behaviors, and interactions observed in complex systems often stem from the information encoded within the system or introduced by an external intelligence. In biological systems, genetic information encoded in DNA serves as the blueprint for the assembly and functioning of organisms. This information cannot be the result of evolutionary processes, because since Darwinian evolution started with the first living self-replicating cells. The discussion of evolutionary processes in the context of genetic information assumes the existence of life and the subsequent diversification and adaptation of organisms over time. Similarly, in synthetic systems or engineered materials, a programmer or designer imparts specific instructions, rules, or algorithms to guide the self-assembly or behavior of the components. Information, in the form of genetic code, algorithms, or predefined rules, plays a crucial role in shaping the behavior and outcomes of self-assembly and self-organization processes. Without the input of intelligent design or programming, the emergence of complex structures or organized behaviors is unlikely, if not, or rather impossible to occur spontaneously. The presence of information or programmed interactions does necessarily imply the involvement of a conscious or deliberate programmer.
The Last Universal Common Ancestor (LUCA): What was its nature?
Before we can start investigating the course of evolution, we need to know what the starting point was. A lot has been speculated regarding the first life form. What did it look like? Was it indeed a Last Universal Common Ancestor (LUCA), or did life start polyphyletic? I have dedicated an entire chapter to my previous book: On the Origin of Life and Virus World by means of an Intelligent Designer, attempting to get closer to answering what could serve as a model organism. This is a surprisingly difficult question to answer.
The last universal common ancestor represents the primordial cellular organism from which diversified life was derived. It has been considered as the branching point on which Bacteria, Archaea and Eukaryotes have diverged.10
Carl R. Woese (2002): The central question posed by the universal tree is the nature of the entity (or state) represented by its root, the fount of all extant life. Herein lies the door to the murky realm of cellular evolution. Experience teaches that the complex tends to arise from the simple, and biologists have assumed it so in the case of modern cells. But this assumption is usually accompanied by another not-so-self-evident one: namely that the ‘‘organism’’ represented by the root of the universal tree was equivalent metabolically and in terms of its information processing to a modern cell, in effect was a modern cell. Such an assumption pushes the real evolution of modern cells back into an earlier era, which makes the problem not directly addressable through genomics. That is not a scientifically acceptable assumption. Unless or until facts dictate otherwise, the possibility must be entertained that some part of cellular evolution could have occurred during the period encompassed by the universal phylogenetic tree. There is evidence, good evidence, to suggest that the basic organization of the cell had not yet completed its evolution at the stage represented by the root of the universal tree. The best of this evidence comes from the three main cellular information processing systems. Translation was highly developed by that stage: rRNAs, tRNAs, and the (large) elongation factors were by then all basically in near-modern form; hence, their universal distributions. Almost all of the tRNA charging systems were in modern form as well. But, whereas the majority of ribosomal proteins are universal in distribution, a minority of them is not. A relatively small cadre is specific to the bacteria, a somewhat larger set common and confined to the archaea and eukaryotes, and a few others are uniquely eukaryotic. Almost all of the universal translational proteins (as well as those in transcription) show what is called the canonical pattern, i.e., the bacterial and archaeal versions of the protein are remarkably different from one another, so much so that their difference is distinguished as one of ‘‘genre’’. Except for the aminoacyl-tRNA synthetases the corresponding eukaryotic versions are virtually all of the archaeal genre. Why canonical pattern exists is a major unanswered question. In the overall it would seem that translation, although highly developed at the root of the universal tree, subsequently underwent idiosyncratic modifications in each of the three major cell types. Transcription seems to have been rather less developed at the root of the universal tree. The two largest (the catalytic) subunits of the DNA-dependent RNA polymerase are universal in distribution.
The cell is the essence of biology. At least that is how 20th-century molecular biology saw it, and the great goal was to understand how cells were organized and worked. This goal, it was assumed, could be accomplished by cataloging (and characterizing) all of the parts of the mechanism, with the tacit assumption that given such a parts list the overall organization of the cell would become apparent. Today, such lists exist for several organisms. Yet an understanding of the whole remains as elusive a goal as ever (34). The fault here lies with the reductionist perspective of molecular biology. The problem of cellular design cannot be fit into this rigid, procrustean framework. It should be obvious from the foregoing discussion that biological cell design is not a static, temporal, or local problem.
The Dilemma of Cellular Evolution.
Evolving the cell requires evolutionary invention of unprecedented novelty and variety, the likes of which cannot be generated by any familiar evolutionary dynamic. The task can be accomplished only by a collective evolution in which many diverse cell designs evolve simultaneously and share their novelties with one another; which means that
(i) HGT (and a genetic lingua franca) is a necessary condition for the evolution of cell designs, and
(ii) a cell design cannot evolve in isolation; others will necessarily accompany it.
Comment: That sounds suspiciously like a special creation. Once Woese admits that many diverse cells evolved simultaneously, he departs from the concept of universal common ancestry and resorts to polyphyly, that is the proposition, that at the beginning, there was a population of diverse cell designs, each one different from one another, that began to interact through horizontal gene transfer.
Woese continues: There is an inherent contradiction in this situation. Although HGT is essential for sharing novelty among the various evolving cell designs, it is at the same time a homogenizing force, working to reduce diversity. Thus, what needs explaining is not why the major cell designs are so similar, but why they are so different. This apparent contradiction can be resolved by assuming that the highly diverse cell designs that exist today are the result of a common evolution in which each of them began under (significantly) different starting conditions. [Initial conditions do not necessarily damp out for complex dynamic processes; indeed, they can lead to vastly different outcomes. 14
E. V. Koonin (2020): The last universal cellular ancestor (LUCA) is the most recent population of organisms from which all cellular life on Earth descends.
Comment: Koonin goes with the same line of argumentation. He hypothesizes LUCA as a population of organisms. Where did it descend from? A population has to originate from self-replication, which produces offsprings.
Berkley University's website on evolution claims: The ability to copy the molecules that encode genetic information is a key step in the origin of life — without it, life could not exist. This ability probably first evolved in the form of an RNA self-replicator — an RNA molecule that could copy itself. Self-replication opened the door for natural selection. Once a self-replicating molecule formed, some variants of these early replicators would have done a better job of copying themselves than others, producing more “offspring.” 15
Comment: By giving careful examination, such assertions cannot be taken seriously. This is pseudo-scientific storytelling. The evidence does not justify saying that probably, it happened. A self-replicating molecule has never been seen. But also if it existed, it would be helpless to create a living cell. If molecule A self-reproduces n-times we would have AAAAAAA....That is ridiculously trivial and has nothing to do with what we see in a cell. A cell is a cybernetic ultra-complex system, where, thanks to countless concurrent software-driven chemical and physical processes using languages and codes, the material is stored, managed, moved, assembled, converted, and positioned such that the cell survives and self-reproduces. To believe, as proponents of naturalistic mechanisms do, that AAAAAAA... leads to a cell, is like to think that by simply duplicating bricks BBBBBBB... we get a functioning complete self-replicating chemical factory.
Martina Preiner (2020): Many found the metaphor appealing: a world with a jack-of-all-trades RNA molecule, catalyzing the formation of indispensable cellular scaffolds, from which somehow then cells emerged. Others were quick to notice several difficulties with that scenario. These included the lack of templates enabling the polymerization of RNA in the prebiotic complex mixture and RNA’s extreme lability at moderate to high temperatures and susceptibility to base-catalyzed hydrolysis. 16
N. Sankaran (2017): Today, thirty years after the RNA World was first proposed, no one has seen an actual living system that is completely based in RNA. Nevertheless, the hypothesis lives on in the origins of life research community, albeit in a hotly debated, highly contentious atmosphere. Although there are strong opponents, many researchers agree that although far from complete, it remains one of the best theories we have to understand “the backstory to contemporary biology.” Gilbert himself expressed some disappointment that “a self-replicating RNA has not yet been synthesized or discovered” in the years since he predicted his hypothesis, but he remains optimistic that it will emerge eventually. 17
Koonin continues: The reconstruction of the genome and phenotype of the LUCA is a major challenge in evolutionary biology. Given that all life forms are associated with viruses and/or other mobile genetic elements, there is no doubt that the LUCA was a host to viruses.
E. V. Koonin (2017): The entire history of life is the story of virus–host coevolution. Therefore the origins and evolution of viruses are an essential component of this process. A signature feature of the virus state is the capsid, the proteinaceous shell that encases the viral genome. Although homologous capsid proteins are encoded by highly diverse viruses, there are at least 20 unrelated varieties of these proteins. A comprehensive sequence and structure analysis of major virion proteins indicates that they evolved on about 20 independent occasions. 18
Viruses and the tree of life (2009): Viruses are polyphyletic: In a phylogenetic tree, the characteristics of members of taxa are inherited from previous ancestors. Viruses cannot be included in the tree of life because they do not share characteristics with cells, and no single gene is shared by all viruses or viral lineages. While cellular life has a single, common origin, viruses are polyphyletic – they have many evolutionary origins. Viruses don’t have a structure derived from a common ancestor. Cells obtain membranes from other cells during cell division. According to this concept of ‘membrane heredity’, today’s cells have inherited membranes from the first cells. Viruses have no such inherited structure. They play an important role by regulating population and biodiversity. 6
Comment: Since viruses are polyphyletic, and, according to Woese, many diverse cell designs evolved simultaneously, which clarifies the picture: Life arose multiple times independently, and so did viruses. The hypothesis of universal common ancestry is not supported by the evidence. Separate origins of different life forms, and viruses, are.
There is no scientific consensus about LUCA's nature
D. C. Gagler et.al., (2021): Life emerges from the interplay of hundreds of chemical compounds interconverted in complex reaction networks. Some of these compounds and reactions are found across all characterized organisms, informing concepts of universal biochemistry and allowing rooting of phylogenetic relationships in the properties of a last universal common ancestor (LUCA). Thus, universality, as we have come to understand it in biochemistry, is a direct result of the observation that all known examples of life share common details in their component compounds and reactions. 20
Eugene V. Koonin (2020): Considerable efforts have been undertaken over the years to deduce the genetic composition and biological features of the LUCA from comparative genome analyses combined with biological reasoning. These inferences are challenged by the complex evolutionary histories of most genes (with partial exception for the core components of the translation and transcription systems) that involved extensive horizontal transfer and non-orthologous gene displacement. Nevertheless, on the strength of combined evidence, it appears likely that the LUCA was a prokaryote-like organism (that is, like bacteria or archaea) of considerable genomic and organizational complexity. 21
J. D. Sutherland (2017): The latest list of genes thought to be present in LUCA is a long one. The presence of membranes, proteins, RNA and DNA, the ability to perform replication, transcription, and translation, as well as harboring an extensive metabolism driven by energy harvested from ion gradients using ATP synthase, reveal that there must have been a vast amount of evolutionary innovation between the origin of life and the appearance of LUCA. Many of the inferred proteins in LUCA use FeS clusters and other transition-metal-ion-based co-factors. 22
Life started complex
Life had to start complex because the earliest known cells, including a supposed last universal common ancestor (LUCA), were already functionally and genetically complex. The simplest cells available for study have a teleonomic apparatus so powerful that no vestiges of truly primitive structures are discernible. The LUCA was sophisticated, with a complex structure recognizable as a cell, and had representatives in practically all the essential functional niches currently present in extant organisms. Even the simplest known cellular life forms possess several hundred genes that encode the components of a fully-fledged membrane, the replication, transcription, and translation machinery, a complex cell-division apparatus, and at least some central metabolic pathways. Therefore, life did not start as a primitive or simple organism, but rather as a complex entity capable of metabolism, genetic replication, and maintaining a boundary that separates the cell from its environment.
J.Monod (1972): The simplest cells available to us for study have nothing "primitive" about them. They have a teleonomic apparatus so powerful that no vestiges of truly primitive structures are discernible. 23 Elsewhere, Monod stated: ‘We have no idea what the structure of a primitive cell might have been. The simplest living system known to us, the bacterial cell… in its overall chemical plan is the same as that of all other living beings. It employs the same genetic code and the same mechanism of translation as do, for example, human cells. Thus the simplest cells available to us for study have nothing “primitive” about them… no vestiges of truly primitive structures are discernible.’ Thus the cells themselves exhibit a similar kind of ‘stasis’ in connection with the fossil record.
J. A. G. Ranea (2006): We know that the LUCA, or the primitive community that constituted this entity, was functionally and genetically complex. Life achieved its modern cellular status long before the separation of the three kingdoms. we can affirm that the LUCA held representatives in practically all the essential functional niches currently present in extant organisms, with a metabolic complexity similar to translation in terms of domain variety. 24
Last edited by Otangelo on Fri Jul 07, 2023 8:44 pm; edited 11 times in total